– Welcome, everyone, to Wednesday Nite @ the Lab. I’m Tom Zinnen, I work here at the UW-Madison Biotechnology Center. I also work for UW-Extension Cooperative Extension, and on behalf of those folks and our other co-organizers, Wisconsin Public Television, Wisconsin Public Radio, the Wisconsin Alumni Association, and the UW-Madison Science Alliance, thanks again for coming to Wednesday Nite @ the Lab. We do this every Wednesday night, 50 times a year. Tonight it’s my pleasure to introduce to you Julia Nepper. She’s born in Wilmington, North Carolina, and was homeschooled there throughout her entire life. She then went to University of North Carolina at Wilmington and studied biology and chemistry, worked for a year at the University of North Carolina at Chapel Hill, and then came here to get her PhD in biophysics. And she just got done this morning. [laughter] Oh, no, last Thursday. Last Thursday. [laughter] So this is Dr. Julia Nepper. [applause] And she gets to talk to us about one of the more interesting things out there to me: biofilms. There’s nothing quite better than slime. [laughter] And very interesting title: “Unraveling Bacterial Biofilm Development from the Outside In.” So please join me in welcoming Julia Nepper to Wednesday Nite @ the Lab. [applause]
– Well, I feel I should point out I wasn’t actually homeschooled my entire life. There were occasional forays into public school. But, yes, for the most part, Tom, you’re correct.
– I was trying to be [inaudible]. [laughter]
– Trying to make me sound more interesting. So, thank you, everyone, for being here to listen to me ramble about biofilms. So I want to talk a little bit about what this title means, kind of some spoilers for what I’m going to be talking about. The reason that I say unraveling it from the outside in is because, as you may or may not know, all cells are surrounded by a cellular membrane composed of lipids or fats. And in my PhD work, I really focused on exploring how certain lipids in the cell membrane would affect the cell’s ability to form biofilm.
So, hence, looking at the outside to see how that affects biofilm formation. And here’s a biofilm here. This is an image that I actually took. It’s not the greatest image. Other people have taken better ones, but I like it. You can see all those little rod-shaped things are individual E. coli bacteria. And this is a biofilm that’s kind of young, just making its way in the world, starting to form some complex three-dimensional structures. So to give you an idea of what I’m going to be talking about today, give you a road map, I’m going to start out giving you an intro to biofilms in E. coli specifically. Although, a lot of these things are applicable to biofilms in many organisms.
Then we’re going to talk about the E. coli cell membrane and the lipids that we find in that membrane. Then I’m going to talk about my PhD work, which is looking at the role of this one lipid, cardiolipin, in E. coli biofilm development. And then we’ll wrap up with some cool stuff like, what does this mean for the average person? Because I know someone is going to ask that, so I put these slides in for you. So, in general, when we study bacteria, we study them in what’s referred to as the planktonic phase. So, basically, what this means is you have a flask, like this, you have some liquid media in it, and you inoculate it with some bacteria. And you’re usually shaking it so that you get uniform oxygenation throughout the culture. And the point of growing the cells like this is that basically all of the cells will be identical in this way. So you’re trying to make the environment that each cell is an identical to each other cell. And that’s really useful if you’re doing, like, bulk measurements of a population of cells.
You want to make sure that if you’re measuring the expression of one particular gene, the measurement that you get from the population will be representative of what you would get from an individual cell. Plus, it’s just easier this way. It’s so much easier this way. But, in nature, because nature likes to be difficult, that’s not how things are. Just in the past few decades we’ve really begun to realize that bacteria are most often found in what are called biofilms. And a biofilm is basically what happens when a single-celled organism decides to get together with a bunch of other single-celled organisms and work together towards the common good. So it’s a multicellular community. And when I’m explaining what a biofilm is to people, I usually like to use rock slime, like you might find in a river, as an example. This is a great example of a biofilm. Biofilms don’t have to be composed of a single species either.
So, for example, rock slime is probably composed of algae, like other single-celled protists or hydra or some sort of things, and bacteria. Bacteria are ubiquitous, as we are coming to understand. And since bacteria are usually formed in biofilms, that means that biofilms are thus also ubiquitous. So you find in the lab where people like me study them. You find them on your skin. Organisms like Staphylococcus aureus, which isn’t always bad. Propionibacterium form biofilms on your skin. You’ll find them in exotic locations, like hydrothermal vents deep in the ocean. You’ll even find them in space now because everything is more fun when you do it in space.
[laughter] And because this is Wisconsin, I have to mention that they’re very important in making cheese. So, why do we care about biofilms in E. coli? When I say E. coli, most people think of, like, this horrible disease-causing organism, which in some cases is true, but generally it’s not. So I want to dispel that stereotype. But there’s also another stereotype that you find among biologists and life scientists that if you’re studying E. coli, you’re just, like what you’re studying isn’t necessarily applicable. It’s like when you find a study that’s like, oh, yeah, we showed this compound can, like, eliminate cancer in rats. E. coli is like the rat. But E. coli is actually relevant to the real world. It’s not just this thing that we use in the lab or this thing that’s going to kill us. It is one of the major species that you’ll find in your gastrointestinal tract.
Gastrointestinal tract is a biofilm-like environment also. It can cause disease in the right instances. So if you get E. coli into your urinary tract, it can cause a urinary tract infection. And it is in fact one of the major causes of UTIs. It’s isolated from up to 85% of UTIs. And, actually, this image I have here is a cross-section of a catheter that was removed from a patient that’s been completed occluded by a biofilm. This is composed of E. coli and Proteus mirabilis and, like, one or two other bacterial species. And, in general, E. coli is a model system for biofilm studies because E. coli is probably the best studied bacterium on the planet. We know so much about it.
It’s easy to grow. It grows quickly. It’s easy to work with genetically. And so all of these also apply to E. coli in a biofilm, which makes it great for just understanding bacterial biofilm development in general. So, how does bacterial biofilm development occur? Well, it’s a complex process, as you can see from this very complicated image. First, cells will attach to a surface. Then, they will, like, begin to colonize the surface. They’ll begin to excrete extracellular matrix components. So that’s largely complex sugars and some proteins. And over days, maybe even weeks or months, you’ll get this large, complicated, three-dimensional structure.
So I’m going to talk a little bit about what we know about each stage of biofilm development in general, looking through the lens of an E. coli cell. So for the initial stage of surface attachment, these extracellular appendages are very important. So, talking about things like flagella, which are used in locomotion, and pili, these kind of hairy things, and also conjugative pili, this fella right here. These help the cell attach to the surface. Flagella actually help the cell find a surface in general. And conjugative pili, in addition to helping cells attach to the surface, can serve other roles in the biofilm, namely in genetic transfer. So that structure is actually used to transfer bits of DNA between cells. And this is also important for antibiotic resistance, if that’s something you’re interested in. This is how different species of bacteria will end up transferring antibiotic-resistant genes between each other.
And the way that we know that these appendages are important for biofilm formation is if you look at this image here. So we can very easily just measure the attachment of a cell to a surface, let it incubate for a certain amount of time in contact with that surface, rinse out any cells that aren’t adherent, and then you can add a stain that’ll basically allow you to quantify the number of cells that have attached. And, normally, E. coli does pretty well, gets a good amount of attachment. But then if you delete these proteins, FimH and FimA, which are key proteins in type 1 pili specifically, you get a severe reduction of biofilm formation. The same when you delete this protein fliC, which is very important for producing flagella. So as biofilms mature, they begin to make an extracellular matrix. This is really the defining feature of a biofilm. Cells in culture don’t have these matrix components. And this is what keeps the biofilm safe from any sort of environmental stress.
Now, the composition of the matrix will vary depending on species or even subspecies. It’ll vary depending on the environmental conditions. But, in general, it’s largely composed of polysaccharides. So in the case of E. coli, these will be things like colonic acid, which is composed of, like, repeating units of five different sugars; cellulose, not just for plants; and, okay, let’s see if I can remember how to say this, this PNAG here. It’s poly-beta-1, 6-N-acetyl-D-glucosamine. [laughter] Bonus points if anyone can remember that at the end of the talk. So we have a lot of those polysaccharides. And you can kind of see here these sheets that are indicated by these tiny little blue arrows are cellulose that’s being formed by this particular biofilm. But then other components, like proteins, are also important in the matrix.
So, in E. coli, these are things like curli proteins and antigen 43. These are proteins that are on the outside of the cell that promote cell-cell adhesion. And they also kind of form these protective little cocoons around the cell. So you can kind of see here that all of these bacteria in this biofilm kind of have their own little nest that they live in. And, of course, there’s a lot of genetic regulation that has to occur for cells to be able to form a biofilm. Genes that have to be activated, genes that have to be repressed. And, depending on which state of biofilm formation you look at, about 10% of the genome is differentially expressed when cells are making a biofilm. And in organisms like Pseudomonas aeruginosa, this is actually a heat map of genetic expression in planktonic cells versus biofilm cells. In a different organism, you can see a lot of differentially genetic expression in that organism.
So, again, what genes are affected by biofilm development will depend on the environmental conditions. But, in general, what you’ll see is initially in E. coli this signaling system called CPXAR will sense when the cells attach to the surface. And what’ll happen from there is generally that you’ll see repression of things that promote motility and enhancement of things that promote production of polysaccharides, cell-cell adhesion proteins, etc. Okay. So if I’m a cell in the middle of this biofilm, I’m not going anywhere. So it doesn’t really make sense to expend a lot of energy in producing a flagellum which is twice as long as my body. So that’s why we see a decrease in motility processes. And all of this leads to a stratification in biofilms. So this is another thing that– It’s another reason why people prefer to study planktonic cells because basically every cell in a biofilm is physiologically distinct. You’ll have sort of patches that are similar, but they’re all slightly different because they’re all in a slightly different micro environment.
So cells that are in the initial, like, colonizers of the biofilm, they’ll have flagella, they’ll be more metabolically active. And then as we get further, deeper into the biofilm, we’ll see smaller cells that are not really trying to produce energy. They’re literally just trying to survive. And they’re producing extracellular matrix components. They don’t have any flagella or motility apparatus. So there are three major biomolecules, right? There’s proteins, there’s nucleic acid, like DNA and RNA, and then there’s lipids. So I’ve told you about proteins and nucleic acid in biofilm development, but I haven’t told you about lipids, which is weird because I think I mentioned lipids at the very beginning. So, what about lipids, you ask me. Great question. There’s not really a lot known about the role of lipids in biofilm development, in E. coli or in other organisms for that matter.
Basically, when I started this study, all that was known about this was that this weird lipid, lipopolysaccharide, it’s a lipid that has this huge, complicated sugar chain attached to it, this helps cells attach to a variety of different surfaces. So if we have, like these two bars here indicate cells that are lacking certain components of lipopolysaccharide, and then the darker shaded bar is the wild-type cells. If we look at the attachment of cells to various different surfaces, we see when lipopolysaccharide is messed up, we get decreased attachment. Okay, well, lipopolysaccharide is just a small part of the membrane. Gram-negative bacteria, like E. coli, like membranes so much that they have two of them: the inner membrane and the outer membrane. And they’re separated by the cell wall, which is a thin layer of a polymer called peptidoglycan. Now, the outer membrane is interesting because the outer leaflet of the membrane has that weird lipopolysaccharide molecule. The other membranes are more typical for what we see in biological membranes. They’re mostly composed of what’s called phospholipids.
They’re called phospholipids because they have this phosphate group in them. So, in E. coli, the three major phospholipids are phosphatidylethanolamine, this guy right here, phosphatidylglycerol, and cardiolipin. Cardiolipin is also kind of strange because it has four hydrocarbon tails, whereas most phospholipids only have two. Another thing that’s strange about cardiolipin is that there are three enzymes that can produce it: ClsA, ClsB, and ClsC. And this is weird because none of them are essential. So why would you bother to have three ways of doing something if you don’t even care if I take them away or not? And that’s basically the question that led to the topic of my PhD thesis. What is the function of these proteins? Why are there three of them? So let’s talk a little bit more about cardiolipin. Cardiolipin is the third most abundant inner membrane lipid in E. coli. But, as I just said, there’s really only three of these lipids, so it’s not hard to be the third most abundant.
5% to 10% of the membrane is composed of cardiolipin. We didn’t really know a lot about cardiolipin prior to me doing this study. What we did know is that cardiolipin would increase as cells reach what’s called the stationary phase. So when cells are growing in a liquid culture, when you first inoculate the culture, you have what’s called the lag phase where the cells are kind of just, like, waking up and they’re like, well, what’s going on? And then they realize that there’s lots of nutrients around and so they start growing. A lot. And you get the exponential growth phase. That proceeds for a while and then they basically exhaust the nutrients that are in the media, and we get stationary phase where we’re not getting any death but we’re not getting any growth. And in this phase, we see an increase in cardiolipin. We still don’t know why. But, as I mentioned, these planktonic phases are distinct from the biofilm state.
And no one had looked at how lipid composition changes in a biofilm of E. coli before. So I did. And what I found is that, actually, cardiolipin increases when cells are in a biofilm state. So it seems like cardiolipin has something to do with biofilms. And, sure enough, if I looked at biofilm formation directly, then I see a reduction. So, again, we used a simple assay where we grow these cells statically, no shaking, in these wells. We allow them to attach for a certain amount of time. You wash out any cells that haven’t attached, and then you stain them with this pretty purple stain. If I delete any combination of the three enzymes that produce cardiolipin, then I get a significant reduction in biofilm formation. And then I can recover that if I add in a functional form of the protein that I deleted.
So, for example, if I delete ClsA, I get a reduction in biofilm formation. If I add a copy of that protein on a plasmid, then I get an increase. And if I add a copy of the protein that isn’t able to catalyze the synthesis of cardiolipin, then I actually get a decrease in biofilm formation. So it seems that this phenotype that we’re seeing is directly correlated with cardiolipin, and it’s not maybe some other effect of just the protein itself. So, what’s happening here? How is cardiolipin modulating biofilm formation? So, as I said before, we didn’t really know a lot about the role of lipids in biofilm formation before I did this work. So I wasn’t getting any easy answers from just reading the literature and trying to find someone who said, “Oh, well, this other lipid does this in biofilm formation, so maybe mine does that.” But, luckily, I have some great labmates, and one of them pointed out that a few years ago someone published a paper where they showed that if you deplete phosphatidylglycerol, which is a precursor of cardiolipin, so it’s necessary to build cardiolipin. If you delete phosphatidylglycerol, you get a decrease in production of flagella. So you can see that you get a decrease in swimming motility and you get a decrease in the expression of this protein FlhD. FlhD is one of the master regulators of the flagellar [inaudible].
So I thought, well, maybe part of this phenomenon that we’re seeing is because in addition to losing phosphatidylglycerol, we’re losing cardiolipin. And if you recall, I mentioned that flagella are important for biofilm formation in E. coli. They’re important for that first step of attachment. They allow the cells to explore more surface area, if we have an irregular surface. So, like, if we have some weird honeycomb surface, which we totally didn’t just make in a lab, then these cells can start to kind of get a finger hold into those cracks and crevices. Whereas, if it were just a smooth, rod-shaped cell, then it can only access the very top. And it also forms like a nice protective mesh around the cells to help keep them safe from anything in the environment that might want to hurt them. So I looked at flagella production in cardiolipin deficient mutants and I found that, in fact, there is a decrease in flagella production. In wild-type cells, over 90% of the cells will have at least one visible flagellum.
Whereas, in the complete absence of cardiolipin, less than 30% of the cells have at least on flagellum. Great, that’s fascinating, and that’s, like, directly correlated to biofilm formation. And also I see a decrease in swimming motility. So further support that I didn’t just accidentally knock off all the flagella while I was preparing my samples. But why does cardiolipin depletion result in a decrease in flagella production? So here I started looking at envelope stress. So I looked at this for a couple of reasons. One, also, I should say, you don’t need to read all of that. It was just to illustrate that there are multiple envelope stress systems. But what I do want to highlight is that several of these systems downregulate motility when they are active.
And, I reasoned that if I’m disrupting a part of the membrane, then I could be activating a stress system that is related to the membrane. And, in fact, when I looked at the expression of various genes that are regulated by each of these systems, I saw that I did activate membrane stress. Of the five membrane stressed systems in E. coli, I only activated one: the Rcs system. This system has been correlated with biofilm formation before. It’s important in the late stages of biofilm formation in increasing that polysaccharide production. And I found that if I disrupted key members of the Rcs pathway in the context of a Cls deletion, so no cardiolipin, no Rcs, then I can restore biofilm formation. So that seems pretty good. It seems like Rcs activation is what’s causing the phenotype in these mutants. And I also see that some of the motility defects start to go away.
So I see an increase in the production of flagella and less of an increase in swimming motility, but in some cases I do see an increase in that as well. But that left one final and probably the most difficult question that I had to answer. So I had to figure out what about cardiolipin depletion was activating this stress response in these cells. So I thought about it for a while and I thought about it for a while, and I figured that there were two things that were most likely to cause this effect that I was seeing. One was direct… changes in the membrane physical properties. So if I change the composition of the membrane, then I’m going to change the physics of the membrane. So I’m going to change how fluid it is. Or I’m going to change the membrane potential or something like that. And one was indirect.
I could be changing the ability of these cells to translocate proteins. I’m going to talk about that more in a minute. But, first, I’m going to talk about what direct effects I could be having on these cells that could be causing this phenotype. So it’s been shown that having cardiolipin in an in vitro system increases membrane fluidity. As you add more and more cardiolipin, you get a higher diffusion coefficient in model systems, which correlates with membrane fluidity. Membrane fluidity can be important for a number of things. If the membrane is too stiff, you can’t get confirmational changes in proteins that could be important for, you know, producing polysaccharides or for producing ATP for the cells, for energy production. But someone had already thought about this and they’d already looked at membrane fluidity, and what they found was that there wasn’t really an effect. So in the wild-type, the membrane fluidity is some number, and then, if we delete cardiolipin, the membrane fluidity is some slightly different number but very similar.
So I didn’t think that this was responsible for the activation I was seeing. Another observation that I’d made much earlier was that I decreased ATP production when I depleted cardiolipin. So ATP is basically the energy source for the cells. That’s what they do, used to carry out the majority of cellular processes. And ATP production depends on what’s called the membrane potential. So, basically, you can think of the membrane as a battery. The cells maintain a voltage across the membrane that can power this protein complex to make ATP. And so I thought, well, I’m seeing less ATP, maybe it’s because the battery is broken. But someone looked at that and the battery seems to be fine.
So in wild-type, again we have some number for membrane potential, and if we get rid of cardiolipin, the number is pretty much the same. There are other things, other physical properties that could be changed by deleting cardiolipin, but they’re all really hard to measure in living cells. So I didn’t think it was worth looking at those. So I turned to the second hypothesis I had, that there was a translocation defect in these cells. And things are about to get a little complicated. So hang on tight. Okay, to understand why I thought that this was happening, we have to understand a little bit more about the Rcs pathway. Namely about this protein RcsF. Basically, what RcsF does is it monitors the condition of the outer membrane of the cells.
So under normal conditions, RcsF is shuttled to the outer membrane, and it’s assembled into a complex with this other protein, which is called OmpA. And what this does is it keeps RcsF busy. It keeps RcsF from interacting with this other protein that’s in the inner membrane, which is called IgaA. And what that protein does is it represses the activation of the Rcs pathway. So if everything is good, RcsF is kept busy by OmpA. It doesn’t activate the pathway. But if something happens to disrupt RcsF on its way to its rendezvous with OmpA, then it can bind to IgaA, and it relieves the inhibition of the rest of the pathway. So what that meant to me is that if there was a problem in getting proteins across the inner membrane, then there would be a problem with RcsF and there would be a problem with the Rcs pathway. And what’s responsible for the majority of translocation of proteins across the inner membrane in E. coli is this complex called the Sec translocon.
So most proteins that have to either be inserted into the inner membrane or have to get in between the membranes or have to get to the outer membrane will go through this complex. And it’s been shown before that cardiolipin and the Sec complex are related. So, for example, if I purify this complex from live cells in the presence of a strong detergent, the detergent will remove lipids that are bound unless they’re very tightly bound, for example in the case of cardiolipin. I apologize, this TLC plate is not great. Blame the authors. So here we purify this complex in the presence of a very strong detergent. We see other lipids, like phosphatidylethanolamine, are removed, whereas cardiolipin is still bound. It’s been proposed that maybe there’s a binding pocket here in the structure of the protein where cardiolipin could be nestled sort of stabilizing the protein. Another thing is cardiolipin stimulates the activity of this protein SecA.
And that’s the protein that actually pushes the proteins through this translocon complex. And if we just look directly at the translocation of a protein through the complex and how efficiently that process is carried out, we see that in the presence of cardiolipin this premature OmpA protein is translocated more efficiently. So here we see this is in the presence of just purified E. coli phospholipids. So anything that is in the membrane is in this mixture, including cardiolipin. And here artificial system with just the two main phospholipids, phosphatidylethanolamine and glycerol, no translocation. But then we add cardiolipin and we start to see translocation of that premature protein. So this led me to believe that in the absence of cardiolipin, I would get decreased translocation of this protein that’s important for keeping RcsF from activating the Rcs phosphorelay. And so I looked at protein translocation in these mutants, and what I found supported this hypothesis. So, luckily for me, there is already a great assay for looking at protein translocation.
There’s this protein, alkaline phosphatase, which has to be exported through this Sec translocon to be active. And so you can use its activity as a measure of protein translocation activity. And what I found is that if I induced the production of this alkaline phosphatase in wild-type cells Mg1655, then I would see an increase in alkaline phosphatase activity the longer that I had production of the protein. Whereas, in the absence in cardiolipin, I got negligible production of any active phosphatase, even after two hours. And that’s very similar to what I saw in a mutant that is missing a key component of the Sec translocon. So, to me, what that meant is that the missing link that I had in this pathway was this deficiency in Sec translocation. So to sum up, what I found is that if I deplete cardiolipin in E. coli, then I activate– Wait, why is this– Okay, this is doing something weird. So I apologize for that. I don’t know what’s going on.
Okay, but either way, I activate– If I deplete cardiolipin in this system, then I get a decrease in translocation of proteins. And that causes activation of the Rcs pathway. And that causes a defect in motility and a defect in biofilm formation. So what about other organisms? Because, unfortunately, E. coli is not the only bacterium in the world even though it’s my favorite. So, recently we’ve started to see more research into the role of lipids in biofilm formation. We’ve seen that there’s an extensive lipid remodeling that happens when pseudomonas aeruginosa forms biofilms. This is an important pathogen often found in the lungs of cystic fibrosis patients. Mycobacterium tuberculosis, which causes tuberculosis, uses mycolic acid in its extracellular matrix. This is another lipid that is specific to mycobacterium species.
In listeria monocytogenes, which can be a foodborne pathogen, we see that these interesting lipids that actually have branches on their tails become important as cells are forming biofilms, and we see an increased amount of those. And we see in Staphylococcus aureus that cells modulate their membranes to have decreased fluidity in a biofilm state. So is cardiolipin important? I think yes. So there are certain bacteria species, such as Salmonella enterica, Shigella flexnori, Yersinia enterocolitica, all pathogens. These pathogens are very closely related to E. coli, and, in fact, you can find in their genomes sequences that are almost identical to the sequences for the cardiolipin synthase genes in E. coli. So I think it’s likely that this phenomenon that I’m seeing in E. coli is also applicable to other species. Even something as distantly related as Streptococcus pneumoniae, which it was actually shown in 2008 that if we knock out the cardiolipin synthase in that organism, we get a reduction in biofilm formation by half. So someone else should look into this. If you know anybody, let me know.
But, also, there’s some things about biofilms that I didn’t tell you, which is that not all of them are attached to a surface. We like to think about them attached to a surface because it’s easier that way, but there are such things as aggregates, which are just sort of free-floating, like in the upper left. That is a pseudomonas aggregate. We have things like sludge granules. So you see these a lot in, for example, wastewater treatment where cells will sort of form these aggregates around individual particles of the sludge. And then there’s things like what are called pellicles. So these form on the surface of a liquid. If anyone drinks kombucha, that’s a pellicle, which is on the top of the kombucha. And these don’t have to be attached to a surface.
They can just start in the middle of liquid. So… What I showed indicates that cardiolipin is important for surface attachment, but does that mean that it wouldn’t be important in these cases because these are biofilms that aren’t attached to a surface? I don’t know, but I’d like to find out. So what does this mean if you don’t study biofilms and you don’t study E. coli and you really don’t care about cardiolipin? It could mean a path to new antibiotics. So it’s very useful to be able to reduce biofilm formation. Biofilms can be very useful. They can be great for wastewater treatment, for example. But they also be very, very bad. It’s ridiculously hard to kill a biofilm.
They can be up to a thousand times more resistant to antibiotics. They’re resistant to pretty much everything. They’re resistant to being dried out. They’re resistant to, you have to scrape them off of things. Like, if you have a biofilm on a wound, they will literally just scrape it off because that’s pretty much the best that we’ve got. So it’s good to be able to reduce biofilm formation in a medical scenario. And infection is a big deal. Up to 10% of hospital patients will get an infection while they’re in the hospital. And with the increase that we’re seeing in antibiotic resistance, for the first time ever last year we– I believe it was last year– we isolated a pathogen in the US that was resistant to every single antibiotic that we have.
And that’s going to become more common. And it’s going to be even worse if that pathogen happens to be in a biofilm. So it could be really useful to have these new targets that we haven’t thought about before for developing antibiotics. But that’s a task for someone else. So I’d like to thank a lot of people in my lab. My PI, Doug Weibel, all of the graduate students who have been in the lab, and my undergrad, Stacy. And, of course, I’d also like to thank the people who have given me money: the NSF, the NIH, the Materials Research Science and Engineering Center here on campus, and the Bill and Melinda Gates Foundation. And, with that, I will take any questions you might have. [applause]
Search University Place Episodes
Related Stories from PBS Wisconsin's Blog

Donate to sign up. Activate and sign in to Passport. It's that easy to help PBS Wisconsin serve your community through media that educates, inspires, and entertains.
Make your membership gift today
Only for new users: Activate Passport using your code or email address
Already a member?
Look up my account
Need some help? Go to FAQ or visit PBS Passport Help
Need help accessing PBS Wisconsin anywhere?
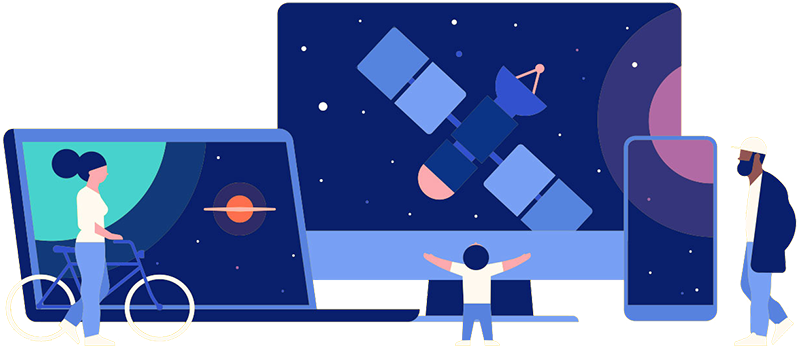
Online Access | Platform & Device Access | Cable or Satellite Access | Over-The-Air Access
Visit Access Guide
Need help accessing PBS Wisconsin anywhere?
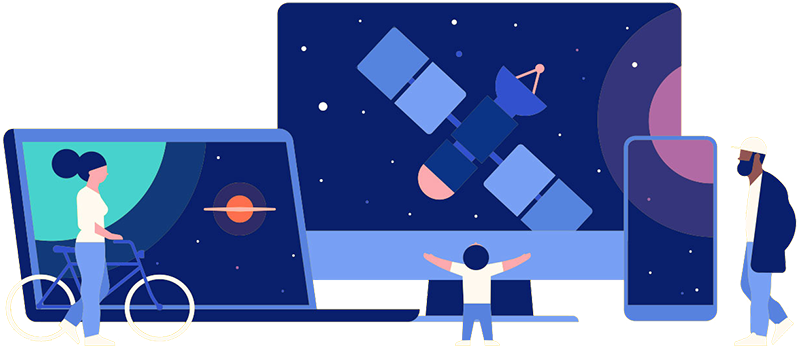
Visit Our
Live TV Access Guide
Online AccessPlatform & Device Access
Cable or Satellite Access
Over-The-Air Access
Visit Access Guide
Follow Us