[Tom Zinnen, Outreach Specialist, Biotechnology Center, University of Wisconsin-Madison]
Welcome, everyone, to Wednesday Nite @ the Lab. I’m Tom Zinnen. I work here at the U.W.-Madison Biotechnology Center. I also work for U.W.-Extension Cooperative Extension, and on behalf of those folks and our other co-organizers, Wisconsin Public Television, the Wisconsin Alumni Association, and the U.W.-Madison Science Alliance, thanks again for coming to Wednesday Nite @ the Lab. We do this every Wednesday night, 50 times a year.
Tonight, it’s my pleasure to introduce to you John Yin. He’s a professor in chemical and biological engineering. He also works at the Wisconsin Institutes for Discovery across the street. Tonight, he’s going to talk with us about C.O.O.L., the Chemical Origins of Life. He’s been working on this since his life began, apparently. That’s how he likes to look at it. And his life began in Boston, Massachusetts. He also grew up for a time in L.A. He went to high school in Kensington, Maryland, did his undergraduate work at Columbia University in New York City, and got his PhD at U.C. Berkeley. Then post-doced in Germany for a while and got his first professor position at Dartmouth College. And then he came here to U.W.-Madison to improve his ice fishing potentials.
[laughter]
I didn’t hear any really cold areas there. This is going to be pretty cool. I love a cube as the central problem of biology.
[slide titled, The central problem of biology, featuring an illustration of a transparent cube filled with a blue lightning-type graphic]
That’s good. A cube does have a center, does it not? Please join me in welcoming –
[Tom Zinnen, on-camera]
– John Yin to Wednesday Nite @ the Lab.
[applause]
[John Yin, Professor, Chemical and Biological Engineering, University of Wisconsin-Madison]
Thanks very much for the kind welcome. Can you hear me all right in the back?
Yes, you can. Very good.
So, I’m delighted to be here. I’d like to thank Tom – Tom Zinnen for inviting me, and it’s really fun to – to be able to share some of our interests. This central problem of biology.
[return to the – the central problem of biology slide with the cube]
This is supposed to be a figure of the universe. So, apparently people who study the universe make simulations of it. And this creates a problem for biology –
[the slide animates on the question – how could purposeful systems have emerged from a universe with no purpose? – in the center of the cube]
– and that problem is perhaps stated here. How could purposeful systems have emerged from a universe with no purpose? This was a question raised by –
[the slide animates on the photo of Jacques Monod and the title of the essay from which the quote appeared – Chance and Necessity: Essay on the Natural Philosophy of Modern Biology, 1970]
– Jacques Monod –
[John Yin, on-camera]
– a famous French biologist and part philosopher, in his work “Chance and Necessity, Essay on the Natural Philosophy of Modern Biology. So –
[return to the – The central problem of biology slide]
– it’s a rather deep philosophical question, I think, and were trying – and we’ll be trying in this next 50 minutes or so to try to answer it a bit more concretely.
[new slide with the words – purposeful systems – on the left-hand side of the slide]
So, how do we do that? Well, we have to say something about what we mean by purposeful systems. So, what I have in mind, and I think what Monod had in mind –
[slide animates on a photo of a cheetah chasing an antelope in the upper left corner above purposeful systems]
– were systems like this. Here’s a jaguar chasing after its prey.
[slide animates on a photo of a Venus Flytrap with its mouths open to the right of the cheetah photo]
Or a Venus Flytrap getting ready for dinner.
[slide animates on a photo of a petri dish full of mold to the right of the photo of the Venus Flytrap]
Maybe some colonies on a Petri dish of mold.
[slide animates on a photo of a snake with its mouth around a frog underneath the photo of the Venus Flytrap]
Or perhaps a snake finding its dinner.
[slide animates on a photo of a spiderweb on a tree branch underneath the words – purposeful systems]
Those aren’t the only things of eating though, here’s a spider web. Probably there’s a spider waiting for its prey. But –
[slide animates on a photo of two squirrels mating underneath the snake photo]
– there are also other systems that have particular purposes –
[laughter]
– such as reproducing.
[slide animates on a microscopic photo of bacteria underneath the photo of the petri dish]
And even bacteria in our gut are reproducing. So, what we mean –
[John Yin, on-camera]
– by purposeful systems here are systems that are alive. They have this property of – of living systems. We can contrast this with purposeless – purpose-free systems. Systems that are not purposeful. What do we mean by purpose-free systems?
[slide with the words – purpose-free systems on the right-hand side of the slide]
[slide animates on a photo of a red rock canyon out West in the upper left of the slide]
Well, ones that are not looking for dinner. Right?
[slide animates on a photo of quartz crystals to the right of the photo of the canyon]
Some minerals, quartz.
[slide animates on a photo of a crescent Moon to the right of the photo of the quartz]
Or the moon.
[slide animates on a microscopic photo of snowflakes underneath the photo of the canyon]
Snowflakes. Beautiful, nice symmetries, but we wouldn’t assign particular purposes to those.
[slide animates on a photo of stars in the night sky to the right of the photo of snowflakes]
Or to the universe.
[slide animates on a photo of a sunset over the ocean underneath the words – purpose-free systems]
Or even to a beautiful sunset.
[John Yin, on-camera]
We’re not looking at systems that try to find dinner here or try to reproduce. And this – in this sense, we might be considering purpose-free systems.
Alright, so, we’d like to think about how living systems might evolve.
[slide titled, How do living systems evolve?, featuring the phrase – simple life – in a gray box with a photo of E. coli above it]
And there is a framework for thinking about this. So, we can consider the simplest forms of life, those of perhaps the friendly bacterium here, E. coli. And the framework for thinking about how this might have evolved –
[the slide animates on a green arrow pointing to the right of the phrase – simple life – to another phrase in a box at the end of the arrow titled – complex life]
– is to think that it moved from simple life, single cellular life, to complex life. We can think of this –
[the slide animates a photo of Albert Einstein above the phrase – complex life]
– as multicellular life. And there’s some process for describing that.
[slide animates on the quote from Albert Einsteins – On the Origin of Species – if it could be demonstrated that any complex organ existed, which could not possibly have been formed by numerous, successive, slight modifications, my theory would absolutely break down. But I can find no such case.]
Elucidating by none other – none other than Charles Darwin, who thought about the various ways that accumulations of slight modifications can proceed. So, if you can’t see it in the back, I’ll read this. “If it could be demonstrated that any complex organ existed which could not possibly have been formed by numerous successive slight modifications, my theory would absolutely break down. But I can find no such case.”
[John Yin, on-camera]
So, realize this was now 1859 in his “Origins of Species.” He had no idea of what we today call D.N.A. So, he’s just thinking about slight modifications that he can observe as expressed in particular biological organisms. But he’s imagining that there’s some underpinning mechanism that can facilitate this, and certainly in the last century this is a major component of finding, at the molecular level, what Charles Darwin was able to observe at the macroscopic level.
So, this is how systems, living systems, evolved. We’d like to try to extend this Darwinian theory from simple life to complex life but now beyond life and extending it backwards.
[slide titled, Extending Darwins theory to chemistry, featuring a smaller version of the previous slide in the upper right corner now with the words – biological phase – above the green arrow and the words – Darwinian theory – below the green arrow]
We might call that a biological phase. If we want to extend it backwards –
[the slide animates the first slide to the left and now includes the words – non-life – in a grey box to the far left and a red arrow from the non-life box to the simple life box. Above the red arrow is the phrase – chemical phase – and below are question marks as to the name of this novel theory]
– we might imagine that prior to simple life there was something we could call a – call non-life and that there was a phase of change from non-life to simple life, which we’ll call a chemical phase. So, the idea here is that that red arrow consists of chemical systems, not biological systems. And that’s what we’re trying to elucidate a bit here.
[slide animates down with a mirroring of the above illustration from non-life to simple life to complex life, but now the chemical phase consists of six smaller arrows one after the other gradating in color from red to green and underneath the illustration is a red line from non-life to complex life that is labeled – Darwinian theory]
So, one way we’ll take to do this is to imagine how we might extend Darwinian theory beyond simple life or complex life to chemical systems. Could we imagine chemical systems that could exhibit biological properties –
[John Yin, on-camera]
– that could evolve? That’s what we’d like to try to do.
So, today we’ll be focusing, then, on this aspect of the –
[return to the – Extending Darwins theory to chemistry – slide]
– the transition from non-life to simple life, and we’ll take a couple of different approaches. One is to start at simple life and try to deconstruct it a bit. See if we can allow it to evolve toward something almost chemical. And then we’ll come from the other side of non-life, simple chemicals, and try to give them, mix them in appropriate ways that they begin to exhibit life-like characteristics. So, Darwin, in his own day, had no, made no attempt to try to describe how simple life would arise. I think he would probably be delighted to see that his theory might have some application beyond –
[John Yin, on-camera]
– living systems. So that’s what we’re trying to do today.
So, we can think of that to the right, everything to the right of simple life as being purposeful –
[return to the – Extending Darwins theory to chemistry – slide, now with everything from simple life to complex life shaded green indicating purposeful life and everything to the left of simple life remaining white to indicate purpose-free and in-between states]
– and everything to the left –
[slide shades the areas of non-life on the slide red to indicate definitive purpose-free life]
– of non-life being purpose free. And I’ll let you decide where purposeful comes in in that transition chemical phase. I’m not going to try to define that tonight.
[new slide tilted, Chemical Origins of Life (COOL) Research, with John Yins titles on it]
So, title of my presentation here is “Chemical Origins of Life.” I kind of like that, C.O.O.L. So, this is the C.O.O.L. research project.
[John Yin, on-camera]
I’m here in the Department of Chemical and Biological Engineering, and I also lead the Systems Biology Theme in the Discovery building.
So, I’d like to start off by suggesting a nice book that I read last September.
[slide featuring the cover of a book titled, What is Life? How Chemistry Becomes Biology by Addy Pross]
This is “What is Life?” by Addy Pross. Some of you might know that the famous physicist Erwin – Edwin Schrodinger also wrote a book by this title. Pross comes at it, though, from a perspective of a chemist, a card-carrying organic chemist. And I think he offers some real insights into how one can be thinking about how biology – how biology could emerge from chemistry.
[slide animates off the book cover and notes the books point that – There are two kinds of stability]
And one of the observations he makes is that there are, well, at least two kinds of stability. He focuses on two kinds. One kind of stability –
[slide animates on the phrase – thermodynamic stability – non-reactive – along with a photo of three rocks]
– is what one could call thermal dynamic stability. It’s non-reactive. It’s like this bottle of water, right? There’s no reaction going on here. Or it could be a few stones there. Nothing happening there. But there’s a different kind of stability –
[slide animates on a new phrase – dynamic (kinetic) stability – highly reactive – along with a microscopic photo of two cells]
– which he calls dynamic or kinetic stability. And this is a highly reactive kind of stable system. That might sound contradictory, so we have to –
[John Yin, on-camera]
– try to unpack that idea of what a dynamic stability could be. Something that’s constantly changing but some-somehow also maintaining something about its behavior. And here I have chosen a figure of a couple of –
[return to the previous slide]
– of living cells here. So, those would be an example of systems that exhibit dynamic stability.
[new slide titled, examples of dynamic (kinetic) stability, featuring a photo of a fountain]
Other examples might be – this is a fountain in Mexico. I was in Mexico last week, and so here, what you see in the white here, are the water, where the water is coming in. And here’s the – the stone or part of the fountain sculpture. And what I’d ask you focus on is the water part. And you have to imagine that this is spraying out and creating this pattern of a couple of areas of water, which appear to be quite stable, but any given water molecule that you see at one instant in time –
[John Yin, on-camera]
– you won’t see for another few seconds, perhaps after it circulates through. Yet the pattern here is quite stable. So, this is one where the water is constantly changing, yet the pattern of the water is maintained. What are other sorts of examples of dynamic –
[return to the – examples of dynamic (kinetic) stability – slide with the photo of the fountain]
– or kinetic stability?
[slide animates on a photo from a cookie factory of cookies on a conveyor belt]
This is a little bit hard to see. I’ve got to find a better picture for this. But you have to imagine kind of a conveyor belt here where this conveyor belt is moving from top to down here. And these objects here are cookies. So, this is a process in – if you go to the cookie factory. Way, way, upstream, up there, is where they mixed up the batter and the sugar and all the ingredients. And they put it on these – on this conveyor belt, and the conveyor belt goes through a continuous oven. It’s got openings at the entrance where the cookies move in, and an opening at the end where the cookies come out. And here they’re starting to – to leave this conveyor belt and then go on to a cooling rack. And this is, I would claim, an example of dynamic stability because if you stare at this and you take pictures of this at every instant, it’ll look something like this. There’ll be different cookies –
[John Yin, on-camera]
– but the same pattern will be reproduced over and over again. If you bake cookies at home, you might be more akin to something like this –
[return to the previous slide now with a photo of a woman placing cookies in the oven and the phrase – A B_ _ _ _ _ of cookies superimposed over the photo]
– where you only have one door. And we might call this a certain kind of – what do we call this for cookies?
[several audience members, off-camera]
Batch.
[John Yin, off-camera]
Batch. B-A-T-C-H. That is not an example –
[slide animates a big red X over the top of the photo of the woman baking cookies]
– of dynamic kinetic stability. We put the batch into the oven. It’s constantly changing. When we peek in the oven at different times, we see it’s brown to different extents. So, over time, that is not an example of dynamic kinetic stability. What might be another
[slide animates on an illustration of the human body under the photo of the conveyor belt cookies]
– Oh, this one. So, you look at me up here and you might think this guy is – how is this guy an example of dynamic kinetic stability? Well, I do have to inhale in order to speak.
[slide animates in the words – oxygen – with a green arrow pointing towards the human illustrations mouth and the words – carbon dioxide – with a grey arrow pointing away from the human illustrations mouth]
So, as I am inhaling. I’m taking in oxygen. Fortunately, there’s oxygen in this room. And as I exhale and speak, I’m producing these words for you. So, I am also a dynamic –
[John Yin, on-camera]
– stable system. Some of my family members may – may have some questions about the stable part.
[laughter]
But I am taking in – taking in the oxygen and producing carbon dioxide. So, I too am an example of a dynamic stable system.
Now, I come from the area of chemical engineering, so I have to teach you at least a little bit about chemical engineering. So, this would be an example of a – of a reactor –
[new slide still under the label of – examples of dynamic stability – now featuring an illustration of a continuous stirred-tank reactor]
– which we call a continuous stirred-tank reactor. It’s stirred tank because we’ve got a little mixer here, and you can think of that as a propeller. And it – when that propeller is spinning around, that means everything in the tank is well mixed and of – of the same composition. This tank also has some feed here. Those of you who might be from the more quantitative sciences might see something that looks like changing of velocity with time. So, this would be a volumetric flow rate. So many – so many gallons per hour might be the flow rate into this vessel. And this might be also at some concentration of some component, concentration in. And then within the tank we have a concentration of that component. Maybe it’s sugar within the tank. And then there’s a volume of the fluid in the tank. And then coming out there’s some flow rate – flow rate of – volumetric flow rate coming out. Now, if the flow rate coming out is the same as the flow rate going in, the volume within the tank will not change. And this will be an example of dynamic stability, in the sense that the volume is constant even though the contents –
[John Yin, on-camera]
– of the tank are constantly being renewed and being drawn off. Notice that the composition of what comes off –
[return to the – examples of dynamic stability – slide with the illustration of the reactor]
– will always reflect whatever the composition is – was in the tank. If we take it even another step further for the mathematically inclined –
[slide animates on the equation dX divided by dt equals kMX minus gX, where X is the concentration of the replicator time variable, k is the rate of replicator formation, g is the rate of replicator decay, and M is the food for the replicator]
– we can try to think about things that might grow in the tank. So, if it’s sugar water, I might introduce some bacteria in there, and the bacteria might grow or the yeast might grow, if I’m interested in – in fermentations. So, we can call those bacteria or yeast replicators. That’s a more generic term. Something that replicates or grows. And one way we might represent how that concentration of replicator changes as a function of time in here is this is a term called a derivative (d). Something we teach our – our undergraduate students in calculus. And we’re saying how rapidly or how this concentration changes depends on two kinds of terms. Terms of growth, and the growth terms depend on how much replicator we already have in there as well as what is this M. This M is the food. Concentration of food. If we have zero food in there, there’s no growth. But if we have some non-zero positive value for M. This is positive. And k is a rate of replicator formation given the presence of replicators and food. So, all of those are positive values. Likewise, g is a positive value. It’s a rate of decay or death. And that would include not only if these cells were dying in here, but also it would include some kind of accounting for how they’re leaving the tank. So that g combines both those kinds of terms. g is positive –
[John Yin, on-camera]
– and X is positive. So, the term minus gX would be a negative term. And what happens in cases of dynamic stability? Then this equation simplifies. Do we have any mathematicians or engineers in the audience, or physicists, perhaps some chemists, who could tell me how this equation would simplify?
[audience member, off camera]
dX/dt will be zero.
[John Yin, on-camera]
dX/dt will be zero. Thank you. Let’s hear an applause.
[applause]
I want you in my class.
[laughter]
Alright, so that means that’s zero, and if that’s zero –
[return to the – example of dynamic stability – slide now with the dX divided by dt crossed out and replaced by a zero in the equation]
– then that means we have a balance between the growth and death –
[slide animates on a green arrow above the kMX part of the equation that is labelled – growth – and a grey arrow above the gX part of the equation that is labelled – decay]
– or we have a balance between growth and decay. Those are equally balanced, and that means if we sit and look in this tank at any given point in time, there is no change in the concentration of our replicator, even though it is growing and it also dying. It’s just that those are balanced –
[John Yin, on-camera]
– quantitatively balanced.
I could take it a step further and put tank inside of a process. Don’t look at the details here –
[return to the – examples of dynamic stability – slide now with an illustration of the reactor as part of a larger process]
– just realize that there are a bunch of tanks connected together with other things, and there are arrows here. The main thing is in this process there are inputs to the process. This – this happens to be a biomass process. So, you put in biomass, and you get some – you get some – fuel out of this thing. But this – this has inputs and outputs, and if we look at any component within this process, it’ll be like this one in the sense that it’ll be a dynamic stability.
[slide animates on a photo of a large chemical processing plant below the equation]
Likewise, I can look at a big chemical plant where there are many more of these operations, we might call them unit operations, where somewhere in this plant there’s a – theres a feed, there are a bunch of distillation towers, there are a bunch of other processing components, and then there’s some outlet. Essentially, somewhere in here there’s a way to feed in raw materials, and there’s a way to pull out products. And when this plant is running, then it’s also an example of dynamic stability. Alright? So that’s your chemical engineering for the day. Now we need to move on a little bit. And we’ll just emphasize the point, from process perspective –
[return to the slide with the book cover for What is Life? How Chemistry Becomes Biology by Addy Pross, now with a note that Darwins Theory of Natural Selection should extend to replicator chemistry]
– Darwin’s theory of natural selection should extend to replicator chemistry. So now we’re trying to look at the chemistry, and we need to say something a little bit about chemistry.
[new slide titled, and now, just a little chemistry]
So, just a little bit of chemistry. I want to introduce a few terms.
[new slide featuring a photo of several plastic containers of various sizes lined up all in a row and the statement – polyethylene is the most common plastic]
So, one kind of chemical compound that you can find is a polymer or polyethylene. It’s a – its a very common plastic. And you – you can find your ice cream perhaps distributed in polyethylene containers. And I already alluded –
[slide animates on an illustration of small green ovals labelled monomers with an arrow pointing down to an illustration of all the monomers connected together labelled polymer and noting that plastics are polymers made from monomers and the process of changing monomers to polymers is called polymerization]
– to this term, that if you look at the plastic and ask, what is the technical term for the plastic?, you might encounter this term polymer, which is made up of monomers. So, the monomers are single units, and the polymers are stitched together. Chemically stitched together monomers to make what we call this polymer. And the process of chemically linking together the monomers to make this chain is called polymerization. So that’s a little bit of chemistry.
[slide animates on the chemical equation for the polymerization of ethylene and notes that the result is polyethylene]
And the process of polymerization – so, the polymerization of monomers of something called ethylene, which contains two carbon groups, yields a polymer or plastic that we call polyethylene That’s what we had over here. [indicating the plastic containers] In the chemical formulas, you have carbons with hydrogens connected to them. And the polymerization process, which chemical engineers like to do, is to link together these – these monomers to make long chained polymers, and then process those polymers into nice shapes that can be useful.
[John Yin, on-camera]
So that’s polymerization. That’s un-unnatural polymerization. You won’t go into nature and find polyethylene, at least – well, I should be a little careful.
[laughter]
These days – these days you might find some plastic out there, but not naturally formed polyethylene. Perhaps released by some living form. But there are also polymers that exist in living systems.
And here we should –
[slide titled, DNA is a polymer made from 4 unique monomers, featuring the chemical illustrations for the four monomers, Adenine, Cytosine, Guanine, and Thymine as well as their chemical equations]
– say something about D.N.A. You’ve heard of D.N.A. For our purposes, D.N.A. is indeed a polymer and it’s made up of monomers. Not the same single unit of monomer as we see for polyethylene. But now we have four unique monomers. And we won’t go into the chemical details. They have different shapes, and they’re made up of different elements. The purple is a common element shared by them, but the bases, these other colors, represent how they differ. And these are sometimes abbreviated A, T, G and C for these chemical compounds. Adenine, cytosine, guanine, and thymine. We don’t need to worry about those details. The main point is D.N.A. is a polymer. So, you imagine somehow stringing these together. Chemically linking them together.
[John Yin, on-camera]
And chemically linking then I could make something out of solely A or solely C or mixtures of A and C. What is important here is that I can begin to encode information using the different monomers.
I could do the same if I had just monomers of ones and zeros. And that’s what you have in your computer. Ones and zeros essentially encoding information, except they’re not chemical ones and zeros; they’re electronic forms of ones and zeros.
Alright, so this is just to iterate –
[return to the slide featuring all the photos of living things along with a new phrase – ALL life uses]
– or emphasize the point that all life uses the same monomers. So, they’re the same monomers of D.N.A. What differs from the D.N.A. of these various different organisms?
[new slide featuring the phrase – every organism has a genome made of D.N.A. – at the top and underneath are two photos, one of an ear of corn and one of a fertilized human egg, both have blue arrows pointing down to two subsequent photos of a stalk of corn and a photo of Albert Einstein and the phrase – genomes differ in length and sequence – at the bottom]
Well, it all boils down to every organism has its own compilation of total D.N.A., which we call its genome. And the corn plant has a genome. You have a genome that was provided to you by your parents as – when you started off as a fertilized human egg. And that genome then defines the various processes of development that ultimately result in a mature corn plant when these seeds are placed in the appropriate environment, or when this fertilized egg is allowed to develop in the appropriate environment, to complex organism.
Key differences here are that the genomes have different lengths, and they have different sequences. They are chemically identical, though. So that’s pretty remarkable. That tells us we are somehow all –
[John Yin, on-camera]
– all related, all lifeforms.
Okay, so where are we now? We’ve talked a little bit about how we are trying to extend Darwinian theory –
[return to the – Extending Darwins theory to chemistry – slide featuring the shaded areas (red, white, green) from non-life to complex life]
– beyond life to chemical, chemical systems. And we’ve talked a little bit about what sorts of chemicals we might be particularly interested in. Some kinds of polymers that are made out of monomers, where the monomers are different and able to encode information.
[slide titled, how does a virus reproduce, featuring an illustration of the process through which viruses reproduce]
So, let’s take the next step and consider a particular biological system. Recall we’re going to go –
[return to the previous – Extending Darwins Theory – slide]
– and take, start from the simple life here and try to go backwards to something simpler than simple life.
[return to the – how does a virus reproduce? – slide]
So, simple life is a single cell here, but it’s being invaded. And this is a virus particle. So, this touches a little bit on the sort of research we do. The virus particle is a little bit of information. It can be made out of D.N.A. or that information could also be made out of a similar polymer called R.N.A. But the main point is that information is delivered to the cell, and when that information is unpacked in the cell, it can direct the resources of the cell to make more virus. Alright? So, the cell normally, when it’s provided growth media, the cell itself will divide in two. It’ll grow larger and divide in two. When the virus finds such a cell, it sees the cell as raw materials. So, here I am putting on my chemical engineering hat and saying this is actually like that – perhaps like those processes where we have some raw materials being converted into products. Except from the standpoint of the viruses, it’s putting in the information that’s informing the raw materials in this cell how to make viruses.
[John Yin, on-camera]
It’s turning the cell into a virus manufacturing plant. And you might make anywhere from a hundred to a thousand or 10,000 viral progeny from a cell. And this – this could take anywhere from 15 minutes –
[return to the – How does a virus reproduce? – slide]
– to 15 hours or 15 days depending on the virus that you might be working with.
Alright, so this is how a virus reproduces. What we want to do is deconstruct this a bit and reduce it to a little bit of its chemistry. So, I want to take a look at a little bit of what’s going on in the deconstruction and construction of viruses here.
[new slide titled, History of Systems Chemistry, featuring an illustrated detail of how viruses reproduce, with an illustration of virus progenies at the top which gets packaged with proteins and then synthesize with them in a process called translation which creates a virus genome that feeds back on the proteins and replicates – genome replication]
So, this goes back into thinking this – this approach was an early stage of systems chemistry. I would call this the chemistry of figuring out how biology might have come about from chemical processes. So, when a virus enters the cell, I alluded to the virus genome, and this – this is used as a template to make proteins. Proteins then feed back on processes that allow the genome to reproduce itself, and then the genome gets together with these proteins and ultimately makes – packages – packages them up and makes virus progeny. So, this does not show all the resources of the cell –
[John Yin, on-camera]
– but it shows some of the components that are important for the virus.
If we take even a greater simplification and look at this virus genome, then we can ask what – what is chemically happening there. So, this virus genome for a particular virus –
[new slide still under the title – History of Systems Chemistry – now with the subtitle – exponential growth of RNA in a test tube. The slide has an illustration of a test tube with liquid in it that indicates the initial condition of the test tube liquid is a few strands of plus R.N.A. along with an excess of monomers and an excess of replicase. To the left of the test tube illustration is the illustration of the process: there is a single strand of red (normal) plus R.N.A. and some excess monomers, when the virus enters it attaches to the replicase (enzyme) that attaches to the monomers to create two strands of R.N.A. one red (normal) plus strand and one minus blue (infected) strand. These infected plus and minus strands of R.N.A. split and the virus having encoded to the replicase (enzyme) continues to create infected polymers from the excess monomers and continues to create pairs of infected R.N.A.]
– I’ll be considering one called a phage, the virus genome was made out of a piece of R.N.A. So, this is – you think – you should think of this as a polymer made out of D.NA. or R.N.A. like monomers. So different monomers where they have a unique sequence. And this plus strand, plus indicates that there’s a certain kind of polarity. If you can think of – well, we could also think of this as ones and zeros. And the monomers then are components that can be – stick to the R.N.A. and be stuck together, bound together by a particular enzyme or catalyst that the virus brings into the cell. The virus also encodes this enzyme. So, when the virus genome gets into the cell, it instructs the cell to make this enzyme.
And what happens is the enzymes uses the genomic template that the virus introduces into the cell, and it uses monomers that are present in the cell to stitch together a complimentary piece of R.N.A. So, the red was what came in with the infecting virus, and the blue is something that is made within the infected cell. So, you can imagine if we think of monomers –
[John Yin, on-camera]
– as being 1-1-0, the first few monomers here as being 1-1-0, on this opposite strand, coding in the negative sense, you would have –
[return to the – Exponential growth of R.N.A. in a test tube – slide]
– let’s see, I said 1-1-0, so across from 1 I would have 0, and it would be 0-0-1. Alright. So, you can imagine how strings of ones and zeros on the red strand could be complemented by ones and zeros on the complementary blue strand. So, either strand encodes the same information, alright?
And what happens within the cell as these two strands separate during an infection, and the replicase can – then can operate the same way as it did on the red strand to make another blue strand. But the replicase can also now – also now operate on the blue strand to polymerize and make the complementary red strand. So, we started off with a single red strand, we made a red and blue, and now we have two each of the red and blue. And this can continue on. So, you have the possibility for exponential, or you could call it explosive growth.
[John Yin, on-camera]
What the virus does when it enters the cell is it essentially sets off a chemical or a genomic explosion, making many copies of the genome.
So, what happened in the 1960s was a biologist –
[return to the – Exponential growth of R.N.A. in a test tube – slide]
– by the name of Sol Spiegelman said this is a very interesting reaction, I wonder if we could deconstruct it and think of it more in chemical terms. So, what he did was he and his coworkers isolated this red strand from infected cells and the polymerase, this enzyme, and he put them into the test tube, and he bought from the store the monomers. So, chemical warehouse – chemical stores you can buy the monomers and you can also put in there. So, we no longer have this reaction going on during an infection inside of a living cell. He’s trying to reconstruct this process of amplification in a test tube, in a little plastic tube here.
So, he has a few strands of the R.N.A., excess monomers or building blocks that he bought from the store, and excess replicase which he isolated from infected cells.
[John Yin, on-camera]
And the question was, Well, would this run or not? Maybe if you provide all the ingredients for the – the initial strand here and the –
[return to the – Exponential growth of R.N.A. in a test tube – slide]
– the enzyme, the catalyst that stitches together the monomers, and plenty of monomer, then you could keep on doing this process of molecular growth. Would that be possible?
[John Yin, on-camera]
Well, indeed he tried that. This is Sol Spiegelman. And so, at time zero he mixed the ingredients together –
[new slide still under the title – History of Systems Chemistry – featuring a graph of Mr. Spiegelmans results showing time in the x-axis and on one y-axis infective R.N.A. units and the other y-axis R.N.A. concentration – the graphs shows increasing infective units over time]
– incubated them at, I think, about 37 degrees C, about a little warmer than room temperature, and over time he – he was able to measure the concentration of R.N.A. in the tube. You might notice that this – this scale here is on a log scale. So, this is one, that’s 10, and that’s 100. So, the R.N.A. here is – is growing exponentially over time. And this is over a period of two, three hours. It just keeps on growing.
You see initially it starts off with the same slope. So that’s an exponential growth, but then it starts leveling off. And you can imagine that it’s leveling off because it’s using up those monomers. As you use up the monomers, the growth slows down.
So, what he did next was an interesting thing. He took these R.N.A. molecules that were made in the tube, and he introduced them back into living cells. So, normally the virus would introduce these –
[John Yin, on-camera]
– molecules into the cell. Here he – he had a way of introducing them into the cell so the cell could still remain intact. And what he found was the cells would make – would make more viruses. So, he’s taking R.N.A. molecules that were made in the test tube, introducing them into the cell, and the cell now makes viable virus particles that he can take and put on other cells, and they’ll continue to grow, alright? So, he’s made, within the test tube, this molecule –
[return to the previous slide with the graph]
– that is infectious.
[the slide animates on the phrase – R.N.A. made in a test-tube is infectious!!]
Alright. This is 1965. There were no companies like Promega selling these biological enzymes. He isolated it from the infection and was finding, essentially, that he could make something that was infectious.
[John Yin, on-camera]
People called these molecules Spiegelman’s Monster.
[laughter]
Because they’re R.N.A. molecules that in some ways behave like life.
So, he took the next step and said, “Well, what would happen if we – if we didn’t allow them to run out of materials?” How could you do that? Well, he wanted to ask the question. So, this is the – this is –
[new slide still under the heading – History of Systems Chemistry – featuring a photo of Spiegelmans scientific article – An Extracellular Darwinian Experiment with a Self-duplicating Nucleic Aid Molecule]
– a couple of years later. Proceedings of the National Academy of Sciences. An Extracellular Darwinian Experiment with a Self-duplicating Nucleic Acid Molecule. So, this nucleic acid, that’s the R.N.A. Self-duplicating, you – you know how that works now. And this is extracellular. It’s outside of the cell. And there’s, well, potential for evolution maybe. And the key point here is he was asking, What will happen to the R.N.A. molecules if only the demand made on them is the biblical injunction, multiply? So, you want to see, if you give them the resources to multiply, how they will do. And that means that you don’t want them to run out. So how do you keep them from running out?
[John Yin, on-camera]
Well, here’s how they set up the experiment. They set up what is called a serial transfer experiment.
[slide titled, An Extracellular Darwinian Experiment with a Self-duplicating Nucleic Acid Molecule, featuring an illustration of the serial transfer experiment showing nine illustrated test tubes in a row with an arrow pointing to the first test tube indicating the initial conditions of a few strands of plus R.N.A. plus excess monomers and excess replicase. Subsequent test-tubes have excess monomers and replicase but no R.N.A. templates]
There’s the tube initially where they had their goodies of the strands of R.N.A. and the monomers and the replicase, but then they set up a whole series of tubes here that had plenty of the monomers, excess monomers and replicase, but no templates. So, there’s nothing going on in these tubes. You have growth initially in this tube. [indicating the first tube with the R.N.A., monomers, and replicase] And before you’re able to use up all of those monomers, you transfer a small fraction of that tube into the next tube. So, some of those molecules that grew come into this land of excess monomers. You can say this land of milk and honey. They’re ready to replicate.
[slide animates on an arrow indicating that part of the first test-tube is being poured into the second test-tube]
So, you do a serial transfer, and they start to grow. They can grow, again, in this tube just as they did in the first tube, except they’re in this tube with all these fresh monomers. And they’re able to grow and before they grow and use up all those monomers, you transfer them again. So, you’re constantly transferring these molecules, and you imagine those lucky molecules that happen to get transferred from tube to tube, the lucky large molecules, only see all this plentiful monomers. So, what happens then?
Okay –
[new slide still under the title, History of Systems Chemistry, now featuring a graph of the results of the serial transfer experiment by Spiegelman showing the measure of total incorporated monomers on the y-axis and the transfer number on the x-axis and showing a steady increase in R.N.A. production]
– so, here’s what happens. You start off with transfer number 0, and these are the various transfer numbers. As he’s doing the transfer numbers, they’re counting or – or doing an accounting of how much of that monomer has been incorporated into the reaction. So, after one – one or a few transfers, you see there are more and more monomers being incorporated. And up through about nine transfers it seems like each transfer is taking up about the same amount of monomer. But around nine or 10 it seems like there’s a – theres a change in this slope. You’re not continuing along the same pattern. You’re actually going a little bit faster, taking up more monomers, alright? So, the system seems to be changing.
Up here it looks like things have slowed down, but actually that’s a little bit of an illusion. What we don’t indicate here is now he’s started transferring even more frequently because these molecules were taking up the monomers so rapidly, they had to reduce the time that they were allowed to take up the monomers. So, what appears to be slower here is actually even faster growth, alright?
[John Yin, on-camera]
So, something is changing as we are doing this. You have to admit this is basically a chemical experiment. We don’t have any living cells here. These are just – there’s an enzyme, there’s a catalyst, it’s a protein, and these R.N.A molecules, then some monomers.
Now what he did was also, as before, he took these templates and he put them –
[return to the previous slide featuring the serial transfer experiment graphed results]
– back into the cell. So, up through passage number nine, when he put these back into the cell, the cell was infectious. It made plenty of – of virus just as before. So, the monster was intact during those first nine transfers. But by transfer 10, and everywhere beyond, none of those molecules when put back in the cell was able to produce a viable virus. Somehow this molecule, this system, lacked – lost the possibility of – of – of making virus. So, the monster is dead.
But some way there’s a different monster here because it’s growing faster. And what he did was to analyze what was finally coming out here. And when he looked at that molecule –
[side animates on the sentence at the top of the graph – Final molecule is 83% shorter than the starting molecule!]
– he found that it was much shorter. 83% shorter than the original starting material, starting molecule. The starting molecule was about 4000 – 4200 monomers in length, and this was only about 17% of that.
[John Yin, on-camera]
Much smaller molecule, alright? So, smaller but – and able to replicate or reproduce much faster than the original molecule, but now not able to instruct the cell to make virus, alright? It’s lost the essential information needed to make viruses, but it’s gained some capability to replicate very rapidly. That’s pretty neat.
And this, I would argue, is an example where the molecules are doing this selection process. There are mutations that are occurring. The mutations happen to shrink the molecule. When the molecule is shorter, it doesn’t take as long to make it. So, when you’ve got resources and you can grow faster than those that are growing slowly, then you get enriched, and you have a better possibility of being transferred to the next tube than the ones that are reproducing more slowly, alright? So that’s a nice example of Darwinian selection at the molecular level. So that’s the example –
[return to the – Extending Darwins theory to chemistry – slide featuring the shaded red, white, and green areas from non-life to complex life]
– that we just considered. Simple life, deconstructing it, putting it into the test tube, and showing that as long as we can replicate and create perhaps some kind of variation, then things can evolve.
Now I want to take it from this side, a system that is clearly non-life, and see if we can move it in this other direction. How do we do that?
[new slide still under the title, History of Systems Chemistry, featuring an illustration of an experiment by Stanley Miller in 1953 showing a prebiotic ocean liquid feeding into a stopped glass container – named the Oceanic compartment – which is then heated creating water vapor. The water vapor runs up a tube to a larger holding container to which sparks are added to stimulate lightning in an Atmospheric compartment that separates out the elements and then goes down a condenser to cool the gas and then this is sampled, and its composition analyzed. To the right of the illustration is a photo of Stanley Miller in his lab working on this experiment. Above the photo is the sentence – Amino acids can be synthesized under assumed prebiotic earth conditions]
Well, my – my second son is a – coming up to 15 years old. So, he’s in ninth grade. And in his biology book he’s got this nice description of this experiment by Stanley Miller from way back in 1953. Some of you might have heard of this.
The idea was to take what was thought to be a primitive atmosphere. Gases in the primitive atmosphere of Earth had things like water, perhaps methane, CO2, ammonia, hydrogen, nitrogen. No oxygen though. So, the early planet was thought to lack oxygen in the atmosphere. But there was certainly water around, so there’s also water down here. So, the gas is able to communicate or exchange material with the water.
And what Miller did as a graduate student, 23-year-old graduate student at University of Chicago, was to put a spark in here. And when he sparked it, he found that there were compounds that were formed in – in the water. And those particular compounds were amino acids. So, amino acids, it turns out, are – have a special place in biology. Amino acids are the building blocks of proteins. And when I say building blocks, another term for that would be –
[Multiple audience members, off-camera]
Monomers.
[John Yin, off-camera]
Monomers. And there are – so, there are different – Boy, you guys are great.
[laughter]
[John Yin, on-camera]
There are different amino acids. Some of you might know there are 20 some amino acids, typically, that we use to build up the proteins in our hair or skin or muscles or – or tears.
[return to the previous slide featuring the experiment illustration and the photo of Stanley Miller]
We’re – were just chalk full of proteins, but those proteins are all made up of amino acid monomers. And the proteins of all lifeforms are made up also of amino acids. So, this was a huge triumph to go from these very simple gases and to realize that the building blocks of biology could be produced. So, I was very curious to turn the page and see what was next in my son’s biology textbook, and they move onto the next topic.
[laughter]
Okay.
[John Yin, on-camera]
That leaves you in suspense. But it says to me also that maybe this field hasn’t really moved a lot since 1953. And that’s – thats both a challenge but maybe it’s also an opportunity.
So, we’ve begun to think about this a little bit, but we also have some help from some giants in the field to think about, How can we start stitching together these amino acids, maybe to make proteins and maybe to – to move a little bit from non-life in the direction of simple life?
So, if you look at the problem, then you immediately hit a wall, and here’s what the wall is.
[new slide still under the title, History of Systems Chemistry, featuring the chemical equations of two identical amino acids that are added together produce water and a peptide link – shown in a subsequent chemical equation below the initial equations. Between the top equation showing the two amino acid equations and the bottom combined equation are two green arrows – one pointing down indicating condensation and one pointing up indicating hydrolysis. The slide notes that the peptide bond synthesis is disfavored in water]
I have to go a little bit more into the chemistry here. The main point here is I have two amino acids. They’re both the same amino acid. There’s an amine group and there’s an acidic group, or acid group. There’s the acid group and amine group on this other amino acid. And what happens, in order to chemically stitch these together, we have to take a water. You might know water has a chemical formula H2O, and if we steal away H2O from these two, then they can stitch together and form a bond. Notice this has C-C-N, and this has a C-C-N, and if we read all along the line here, we have C-C-N and C-C-N. We just formed a bond here, and that’s called a peptide or amide bond. And in the process of making that bond, we kick out the water. So, the water appears down here.
Now, there’s a problem here in that –
[John Yin, on-camera]
– if you go to your freshman chemistry student and ask, “What direction is this chemistry favored if your amino acids are in water?” If you have lots of water present, then the chemistry favored is in the hydrolysis direction, or moving upwards –
[return to the previous slide with the two amino acids combining]
– rather than moving downwards. Moving downwards is what we want. We want to stitch together these monomers to make a two piece – two – two amino acids joined together. But what is favored in actual chemistry is, in water at least, is a hydrolysis or a lysis or lysing or splitting of a molecule by inserting a water. So, the water gets inserted here to make the two separate amino acids.
[John Yin, on-camera]
So, each of us is very unstable. If you go to the swimming pool and sit there long enough –
[laughter]
– this is what’s going to happen. You will be decomposed –
[return to the previous slide with the illustration of two amino acids combining]
– into your amino acids, as long as there aren’t other bacteria there. But the chemistry at least favors your decomposition back to your monomers. Alright, so this is a problem, and this is a serious problem.
In your body, you routinely stitch this together. And this is –
[John Yin, on-camera]
– happening as we speak. You are digesting, you are making proteins, but the machinery to do this is many orders of magnitude larger than the amino acids. So, something –
[return to the previous slide featuring the illustration of the combination of two amino acids]
– the size of this room is the enzyme that helps to stitch together amino acids of this size. So, a highly evolved, very complex machinery made of both protein and R.N.A. That we don’t have present on the primitive Earth. So, we’ve got to figure out a way of trying to do this chemistry in a much more primitive way.
And that’s where I allude to the giants. One of the –
[new slide with the title, Step 1 to peptide bond – form di-mer complexes in solution, featuring five illustrations of amino acid chemical equations one flowing to the other]
– giants in the field is Bernd Rode. So, he is an emeritus professor now from Vienna, Austria. He’s a theoretical chemist. But he thought, You know, there’s this problem of stitching together the – the monomers, I wonder if we could do it in a simple way by putting in some copper chloride. So, this is a little hard to see. I’m sorry the color is a little washed out here. But the copper here is indicated by this – this little trapezoid here. And the amino acids now are shown by the circles here. So, it turns out that if you put some copper chloride into solution, it forms a nice blue solution, but the coppers have this tendency – well, copper is positively charged. It has a two-plus positive charge. The amino acid could have a little bit of positive and negative charge. It has enough negative charge so opposites attract, and that amino acid may become associated with – with the copper. Not only that, another amino acid might associate with the same copper. So now you have two amino acids, two monomers brought into close proximity. You can think of the copper as kind of a matchmaker for the two amino acids, and they’re sitting down to lunch here.
[laughter]
Now, if the appropriate conditions are such that it’s not too acidic, then these two – these two potential partners jockey around, look at each other in the eye, and realize that they might like to come together. And if they actually come together, if you could remove a water at this point, then you can actually drive the chemistry so that these two monomers come to the same side of the table and form that amide or peptide bond. Okay?
[John Yin, on-camera]
The trick here is that you’ve got to remove the water. So, maybe we’ve just shifted, maybe it’s just a semantic point here, but a key aspect here is that you brought these guys in close proximity.
[return to the previous slide featuring the amino acid/copper experiment]
And the removal of the water is where Rode, I think, was particularly clever. One thing he did was to have very high concentration of sodium chloride, salt, table salt, which, in solution, likes to pull on water. Sodium chloride likes to have water around it. So, it can be stripping away water. These are water molecules around here. But what he also did was leave the tube open to the environment, so the water could just evaporate. So, he said –
[slide animates a pink box around the last chemical equation in the list along with the phrase – evaporate the water]
Alright, leave the tube open to the environment, allow the water to evaporate, and maybe you can get the chemistry to go so now there are some – some di-mers. These are two unit now monomers. We’ll call it a di-mer. So, that allows you to – it shows you how you can go from monomer to di-mer.
And then you can imagine the next step might be, well, if you add backwater, and then these guys, this di-mer can go back into solution. But it can associate over here on the side. So, you could have a di-mer over here, and then the next guy adding here could be either a monomer or a di-mer.
[John Yin, on-camera]
If you go around the whole cycle again, evaporate, then you could form two – two di-mers coming together would give you a tetra-mer, or a di-mer and a monomer coming together would give you a trimer. So, by just going around here, essentially drying –
[return to the previous slide with the amino acid/copper chemical equations]
– and then rehydrating and then drying again, we have a machine to be able to stitch together these monomers.
Here we’ve considered monomers that are all the same. But you can imagine that we can now put in different monomers. Any – any drawn for those natural 20 amino acids. And you could imagine that, depending on their particular chemical affinities for copper, that we might have any array of different kinds of polymers that could be formed from different monomers.
So, we had to try this on our own.
[new slide titled, Step 2 to peptide bond – evaporate the solvent (water) – featuring on the left an illustration of a test-tube with a mix of alanine plus CuCl2 plus water which is then heated to dry at 60 degrees Celsius to form a reactive solid – shown in a separate illustration. To the right are two graphs, both showing time on the x-axis, but the top showing mass of water and di-alanine on the y-axis and the bottom one showing di-alanine yield on the y-axis. The top graph shows water mass decreasing over time while di-alanine increasing over time and the bottom graph shows a steep increase in di-alanine yield in a short period of time with the results labelled di-mer formation]
Back about 10 years ago, I had a masters student, and I said, Why don’t you give that a try and – and mix some amino acid, in this case alanine, with some copper chloride and water? You form this nice blue solution. And let’s just heat it to dry it. So, you bring it to 60 degrees C. You just put in on a block. And you leave it open, so it evaporates. But to make sure we could do some kinds of measurements on what’s going on, I said, Well, let’s – lets weigh this thing. So, here’s the mass of the water. We started off with half a gram of water in there at time zero when we initially mixed this together. And then over a period of, oh, six or eight, what, four to eight hours, took periodic samples, weighed it, and because it’s open to the environment, you can imagine this water level just dropping, dropping, dropping as you’re evaporating, and that’s what you’re seeing right here. Mass of water in there is decreasing.
At the same time, we were sampling that water and doing some analysis, some chemical analysis to say, Do you got any di-alanine in there? And so, the di-alanine axis is over here, and during that drying process as the water is dropping and evaporating, we don’t see any di-alanine. We don’t see any di-alanine after eight or 12 hours, but if we incubate this now 16 hours and then we rehydrate it and analyze, lo and behold there’s a little bit of di-alanine. And if we incubate even longer, there’s more di-alanine. So, this solid here is reacting. That condensation reaction is actually occurring in the solid for these. We’re making polymers in here. We’re not making any polymers over here [indicating the initial solution test-tube illustration], but we are doing that here [indicating the second reactive solid test-tube illustration] as we incubate this solid.
And we don’t have to stop at 20 hours. We could go out to 200 hours, and it keeps on going. If we go out to 400 hours or so, it doesn’t maintain the same pattern. This reminds us that maybe we’re starting to use up those monomers –
[John Yin, on-camera]
– as we saw with the R.N.A. experiment, alright? So, you can imagine other sorts of serial transfer experiments you might want to do.
That’s as far as we went in 2006, but Rode did a series of other experiments that were very intriguing also. What they did was to put in other amino acids. So, histidine is another amino acid.
[slide titled, Single amino acids can catalyze amide bond formation, featuring a screenshot of the webpage for the Journal of Inorganic Biochemistry and the article, The catalytic effect of t and d-histidine on alanine and lysine peptide formation by Daniel Fitz, Thomas Jakschitz, and Bernd Rode]
This has some interesting side chemistry. It’s able to – to become acidic or basic depending on its side. It has some more interesting chemistry than the alanine that we are considering. And the main point here is when you put in that additional amino acid, this particular amino acid, it has a catalytic effect. That means that it accelerates the reaction without being consumed.
[return to the Step 1 to peptide bond slide featuring the amino acid/copper chemical equations]
So, you imagine bringing in that histidine. Somewhere the histidine might associate a little bit over here or over here or maybe in the solid phase. The key point is if I’ve got histidine in that system –
[return to the Step 2 to peptide bond slide featuring the test-tube illustrations and the two graphs]
– then this – this growth curve of di-alanine will be even steeper, will make much more di-alanine in the same amount of time than we made in the absence of histidine.
[John Yin, on-camera]
So, histidine is not being consumed. It’s being – its serving as a catalyst.
Those of you who have studied some biology or biochemistry know that enzymes or proteins that have histidine, oftentimes the histidine will play a catalytic role, that it can actually do some chemistry in the enzymes. So, maybe it’s not surprising that the histidine alone in this particular system could do some chemistry.
What was really surprising, though, was when they took glycine. So, glycine happens –
[slide titled, glycine can catalyze amide bond formation, featuring a scan of an article by Kristoff Plankensteiner, Alessandro Righi, and Bernd Rode titled, Glycine and Diglycine as Possible Catalytic Factors in the Prebiotic Evolution of Peptides]
– to be the simplest amino acid you can imagine. Its – its chemistry is just – it’s side group is just this H, a hydrogen. And inside of you there’s no enzyme. I’ve found no – we’ve got a great biology department, biochemistry department here – no one – none of my biochemistry colleagues can tell me of an enzyme in nature where glycine is active as a catalyst. Alright, it’s boring chemistry, alright?
But here, it’s catalytic. How could that possibly be? So, you’ve got this boring amino acid that normally, in our – in our body, doesn’t do anything interesting, but in this particular scenario can do something interesting. And we –
[return to the Step 2 to peptide bond slide, featuring the two test-tube illustrations and two graphs]
– have some thoughts on what’s going on here because when we look at these solids, we see at least two kinds of solids. One kind of solid looks kind of crystalline, like the – if you pour a little salt out of your saltshaker. It’s kind of crystalline. Another kind of solid is kind of glassy. So, it’s kind of amorphous.
[John Yin, on-camera]
And it looks like melted glass. In that case, it turns out that the reactive solid is more reactive when it’s glassy than when it’s crystalline. And we would hypothesize that when glycine is present, perhaps it acts as a bit of a plasticizer and allows the material to even flow a little bit and do some chemistry. So that’s a guess at this point. We haven’t demonstrated that, but we – we think that even the most boring amino acid, a single monomer, can now be an enzyme, and that’s really quite remarkable.
Okay, so where do you go from there? Well, we’re starting to mix amino acids together and cycle them and – and do all sorts of things. But – and we’ve talked about –
[return to the Extending Darwins theory to chemistry slide, featuring the shaded illustration from red non-life to the white chemical phase to the green simple to complex life]
– how we started from the simple life and move down towards non-life. And hopefully I’ve convinced you that maybe non-life could be moving in this evolutionary direction. Maybe I have to elaborate a little bit more on how that would work. I’ve shown you how non-life, how the monomers can be brought together to make polymers. And I’ve suggested that certain monomers can be catalytic, even the most boring ones. If that’s the case, then you could imagine any set of polymers could possibly be catalytic.
So, let’s assume we have some –
[new slide featuring four illustrations labelled A – di-mer, B – penta-mer, and C – di-mer (which is mislabeled – it should be quatra-mer). The A illustration is of two sets of circles, one two red circles connected by a gray line, one a red circle and a blue circle connected by a gray line. The B illustration is of three sets of five circles connected by gray lines; the first set is green-green-red-blue-green, the second set is green-green-red-blue-blue, and the third set is green-blue-red-blue-blue. The C illustration is of two sets of four circles connected by gray lines; the first set is blue-red-green-red, and the second set is blue-blue-green-red]
– building blocks, and here I’ve shown the building blocks are monomers in several different colors. Red, blue, and green. So, we have – we’ve considered three different monomers. And just imagine that I put in the copper and – and do some chemistry and allow these monomers to form di-mers. We already saw that for alanine, that you could form a homo di-mer made of the same monomers. Or you could imagine maybe in some cases you’d form a hetero di-mer made out of two different monomers. Or if we cycled a few times, maybe you could make penta-mers or even some – I’m sorry. This is mislabeled. This should be – what should this be?
[Audience member, off-camera]
Quatra-mer?
[John Yin, off-camera]
This should be quatra-mer or tetra-mer, right? Okay? So –
[John Yin, on-camera]
– of course, I purposefully did that just to see if you were awake.
[laughter]
Alright, so you could imagine in this soup, depending on how many times one of these molecules has been – gone through the process of being dried out and – and rehydrated, that we might have some di-mers or tetra-mers or penta-mers –
[return to the previous slide now labelled, Evolution of molecular cooperation, featuring the three illustrations, A, B, and C]
– and there might be some variation. There’s no kind of template copying here as we had in the R.N.A. case. Alright, so there’s no template copying. So, you could imagine any number of different kinds of polymers arising. And I just indicated a few variants here.
Now, what’s interesting to think –
[John Yin, on-camera]
– is that maybe this di-mer could be like glycine. It could be a catalyst. And when I say a catalyst, that means maybe if this is present, then it helps to make one of these B – B polymers.
[return to the Evolution of molecular cooperation slide]
So, A could serve as a catalyst to enable B to make – to be made more readily, one of these B molecules to be made more readily, more easily, without being incorporated, but just facilitate that – that chemical synthesis. The same could be said of B. Maybe B, one of these B molecules could help a C, and a C could help an A. That’s the interesting part.
[slide animates on an arrow to the right of A pointing to B and an arrow to the right of B pointing to C, and an arrow to the left of C pointing to A]
If you have the possibility of somehow this loop closing because then if A enhances B and B enhances C and C enhances A, this is a positive feedback. And a positive feedback has a – has a capability then of growing out where these molecules enhance their own synthesis, while the ones that don’t contribute –
[John Yin, on-camera]
– so much to the chemistry are left behind. So, you might imagine the red-red variant here does a little bit better job than the red-blue variant.
[return to the Evolution of molecular cooperation slide]
And it would be perhaps enhanced by the chemistry here and allow certain members of each of these penta-mers, di-mers, or tetra-mers and di-mers to grow out. So here, this is just an arm waving –
[John Yin, on-camera]
– but I’m trying to make the case that it’s plausible at least that we could see some synthesis of polymers, hetero polymers made up of different monomers, enhanced in their synthesis and contributing to the – to the bigger picture of self-replication, okay?
So, I’ve tried to make the case that under different environments –
[return to the Evolution of molecular cooperation slide]
– we might form different monomers, or sorry, different di-mers or polymers, and these might react on themselves under different conditions. If we make things a bit more acidic or change the temperature, you can imagine the chemistry is favoring some molecules over others. So, maybe there’s an opportunity also for adaptation or evolution in this kind of system.
[John Yin, on-camera]
Okay. So, there are a few broader impacts or extensions of what I’ve tried to elucidate here.
[slide titled, Broader impacts and extensions, featuring a number list – 1) Do same study with R.N.A. (to test R.N.A. world hypothesis), 2) Combine R.N.A. and peptide emergence (evolution of ribosomes), 3) Emergence of information from non-biological building blocks. (Evolution by chemicals – life based on non-biological molecules), 4) Rewinding the tape (evolution of alternative histories), 5) Do is all ab initio (computational chemistry – origins of info/life)]
I’ve showed it for amino acids, thinking about how amino acids might come together and become functional, enable their own replication. We’re very interested in also doing this with the R.N.A. monomers. For those of you who follow the biology – I’m sorry, this got a little bit scrambled here. But we’re testing the R.N.A. world hypothesis. Some people believe that R.N.A. was actually the first biopolymer to be formed, and there are good reasons for that. We’re not convinced yet, but we think this might be a way to – to show that or find R.N.A. molecules that are able to contribute to their own replication and evolution.
We could imagine taking the R.N.A. monomers and mixing them with amino acids and doing this kind of cycling and perhaps allowing both kinds of polymers to arise. I mentioned that the machinery that stitches together proteins in – in living systems is very much more complex. That machinery is called a ribosome, and it’s made up of R.N.A. and amino acids or peptides. Peptides being amino acids strung together. So, maybe it’d be possible to evolve that kind of machinery in a test tube, where these kinds of cooperation where there’s a cooperation of R.N.A. and – and peptides or proteins.
You don’t have to stick with the amino acids or R.N.A., the monomers that nature gave us. There are many kinds of fascinating amino acids that do not exist in nature. We’re not restricted to the ones that exist in nature. We could carry out the same chemistry using non-biological monomers. And we imagine that they could conceivably also replicate. So, you could imagine making polymers that are reproducing and are evolving and adapting but not made out of anything that arises in living systems. So, we don’t have to also restrict ourselves to the specific kind of polymerization chemistry that I’ve shown here. There are many kinds of polymerization chemistries. So, one could branch out to things that are not just hydrolysis condensation chemistries.
Alternatively, we could stick with the 20 amino acids, and just say, Let’s repeat the experiment several times and see if we get the same monomers condensing to form the same polymers.
[John Yin, on-camera]
How reproducible is evolution? Or if we do it at a slightly higher temperature, will we get a very different kind of distribution of molecular species? Perhaps so. So, what role does environment play on the evolution? This is that idea of rewinding the tape.
[return to the Broader impacts and extensions slide featuring the numbered list]
We are also very interested in computational chemistry. So, the things that happen in the solution phase, this forming of amino acids coming together with copper, is very well studied. It’s been known how that happens for more than 50 years. So, people who want to try to simulate that behavior –
[John Yin, on-camera]
– in computers have no problem with that. It’s just that when you dry and remove all that solvent and form that solid that’s reactive, that’s a complete unknown, and its a – would be a fabulous frontier, I think, of computational chemistry to try to compute how living systems or information containing systems could arise. So, that’s – thats something we’re also currently working on.
So, I try to make the point here that spreading these ideas of Darwinian selection from living systems to chemical systems might give us some insights into ways that life might have originated from chemistries. I’ll end with this particular quote from Richard Feynman –
[slide featuring a photo of Richard Feynman with the quote below]
“What I cannot create, I do not understand.”
Thank you.
[applause]
Search University Place Episodes
Related Stories from PBS Wisconsin's Blog

Donate to sign up. Activate and sign in to Passport. It's that easy to help PBS Wisconsin serve your community through media that educates, inspires, and entertains.
Make your membership gift today
Only for new users: Activate Passport using your code or email address
Already a member?
Look up my account
Need some help? Go to FAQ or visit PBS Passport Help
Need help accessing PBS Wisconsin anywhere?
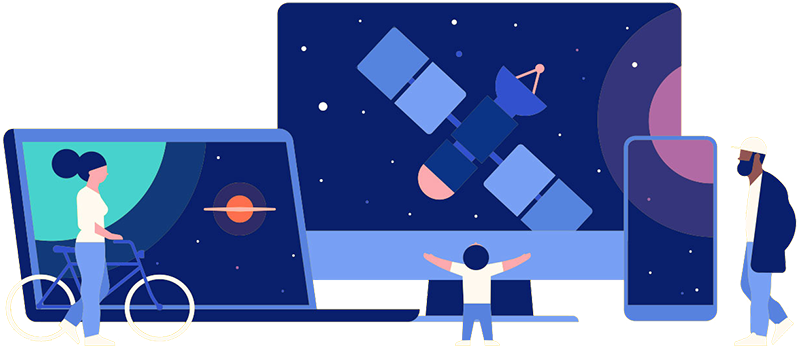
Online Access | Platform & Device Access | Cable or Satellite Access | Over-The-Air Access
Visit Access Guide
Need help accessing PBS Wisconsin anywhere?
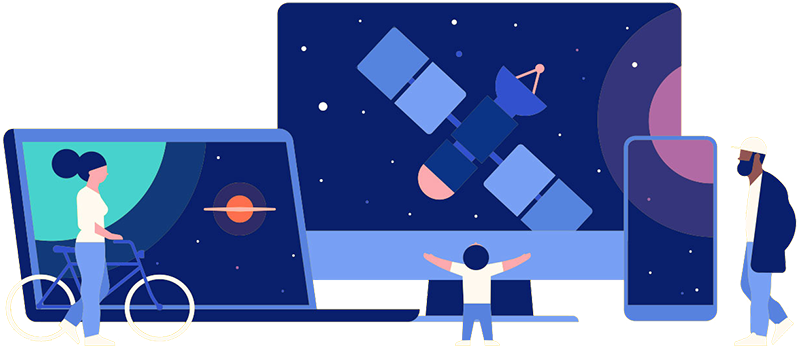
Visit Our
Live TV Access Guide
Online AccessPlatform & Device Access
Cable or Satellite Access
Over-The-Air Access
Visit Access Guide
Follow Us