[Tom Zinnen, Outreach Specialist, Biotechnology Center, University of Wisconsin-Madison]
Welcome, everyone, to Wednesday Nite @ the Lab. I’m Tom Zinnen. I work here at the U.W.-Madison Biotechnology Center. I also work for U.W.-Extension Cooperative Extension, and on behalf of those folks and our other co-organizers, Wisconsin Public Television, the Wisconsin Alumni Association, and the U.W.-Madison Science Alliance, thanks again for coming to Wednesday Nite @ the Lab. We do this every Wednesday night, 50 times a year.
Tonight, it’s my pleasure to introduce to you Kate O’Connor-Giles. She’s a professor here in the genetics department in the cell and molecular biology program. She was born in Champaign, Illinois. She grew up in Champaign, Illinois, and graduated from Champaign High School, at which time she went to the University of Illinois at –
[multiple audience members]
– Champaign
[Tom Zinnen]
– Urbana-Champaign.
[laughter]
[Tom Zinnen]
Where I spent two of the most glorious years of my life. Then she went to Washington University in St. Louis and got her PhD. She came here to do a postdoc with Professor Barry Ganetzky, and now she’s on the faculty here. She gets to talk to us tonight about CRISPR, which is probably the single most interesting new technique as far as breadth and how it’s shaking up the wide fields of life science since polymerase chain reaction, at least that’s my take on it. It’s going to be very interesting, as you can see, with the eraser. A very interesting way to edit genes. Maybe one base at a time, maybe one gene at a time. So, please join me in welcoming Kate O’Connor-Giles to Wednesday Nite @ the Lab.
[Kate OConner-Giles, Assistant Professor, Laboratory of Genetics, University of Wisconsin-Madison]
Thank you much.
[applause]
Okay. Thank you very much. Can you hear me? Okay, so, just before we start, how many of you have heard of CRISPR in the media?
[wide shot of audience with multiple hands raised]
Okay. Following it closely?
[wide shot of the audience with only a few hands raised]
A few of you. Okay. Great. Great.
Well, so, as many of you know, it has been a longstanding goal of biologists to be able to do exactly this. To erase and rewrite a – a genomic sequence, okay? And so, this has made – this new CRISPR technique has – has made –
[slide featuring a photo of two magazine covers, the 2015 breakthrough of the year issue of Science magazine and an issue of Nature magazine featuring an article, CRISPR everywhere]
– a big splash. It was the breakthrough of the year in 2015, according to Science magazine. Nature just last month came out with a – an – an issue dedicated to CRISPR called “CRISPR Everywhere.” There was an interesting article called “The CRISPR Zoo” that you might want to read if the topic is of interest to you, of particular interest to you. It –
[new slide featuring eight photos of the uses for CRISPR, one of two fruit flies labelled – making model organisms for studying gene function, one of a luminous fish labelled – pets, one of a mosquito on a human arm labelled – targeting disease vectors, one of a silhouette of assembled dinosaur bones labelled – de-extinct, one of an illustration of two plants whose roots intertwine to make a D.N.A. strand and a picture of two baby pigs labelled – food production, one of a circle split into six slices each with a photo of a candidate for fuel – labelled biofuel, and one of and illustration of two D.N.A. strands next to one another with a section of one removed and pointing to a section of another and labelled – correcting disease-causing mutations]
– really talks a lot about the many, many things that we can potentially use this new genome engineering technology for. And before I go into the details of how it works, I thought that I would just highlight some of the things that it has the potential to affect. And, in many cases, is actually already affecting.
And so, those things include model organism research. So, those are actually mutant flies that we generated using the CRISPR technique. I’ll tell you a little bit more about that later. Correcting disease causing mutations. So, if we can go in and change –
[Kate OConner-Giles, on-cam]
– a disease allele to a wild-type allele, that would be obviously something that would be of – of great potential value for – for human well-being.
Food production. So, we all know about G.M.O.s. This is a way to modify the genomes of – of –
[return to the slide with the eight photos of CRISPR use]
– both plants and livestock in ways that do – in a way that doesn’t involve bringing in foreign D.N.A., okay? So, this may be a potential way to improve our ability to feed the world. De-extinction, which you guys are going to hear about soon. So, people are actually very serious about using CRISPR technology for de-extinction. So, you’ll hear some about, you know, the – the – the – the maybe the more Jurassic Park type cases, like bringing back the wooly mammoth. One of the big –
[Kate OConner-Giles, on-cam]
– leaders in CRISPR is actually extremely interested in this prospect. But there are other things. For example, heirloom tomatoes, other varieties of foods that are no longer available to us that we might be able to take modern varieties, edit them to make them match varieties that are no longer available.
Biofuels. And, really, many, many other things. Pets. This is – this is happening, not in the U.S., but –
[return to the slide with the eight photos of the potential use for CRISPR]
– editing animals. There’s a Hercules dog that has a large muscle mass that was made using CRISPR.
And then targeting disease vectors. And that one I’m actually going to focus on at the end because this one is really of just dramatically increasing in-interest with the Zika virus. And I think that’s the – the coincidence of this technology, and that – the emergence of this – this mosquito-borne disease actually has a real potential to – to change –
[Kate OConner-Giles, on-cam]
– the course of what might happen.
Okay. So, what is CRISPR? So, I’m going to use a – a typing a Word document as an analogy for – for what – what we’re doing with genome engineering. And so, basically, the first step is finding the spot in the genome that you want to edit. Okay? So, you need some mechanism for searching. So, here what I’ve done is I’ve typed germline correctly –
[slide titled, Document editing analogy, featuring a Word document which Ms. OConner-Giles has typed up with the word germline highlighted in yellow and which is spelled correctly twice and incorrectly once]
– twice and incorrectly once. So, I’m trying to find the one time that I typed it incorrectly, and I failed. I found it all three times by typing in germlin. So, I –
[slide animates out those instances of the word germline that are spelled correctly leaving only the incorrectly spelled instance remaining]
– had to revise my search and add the period, and then I found the correct spot. So, I need – I needed precise localization to the spot that I want to edit. And then I need a way to get my cursor in. So, I need to break into that spot so that I can then go in –
[new slide, titled Document editing analogy, featuring the same document enlarged to the area that the highlighted misspelled word is an showing a cursor at the end of the misspelled word]
– and here I’m just showing this magnified. So, I’ve got my cursor in the right spot. I’ve broken into that word –
[slide adds a second magnified line under the previous line now with the highlighted word spelled correctly on that second line]
– and now I can type in the E and correct this. And this is actually really quite analogous to what we’re doing with genome engineering.
[new slide titled, D.N.A. breaks initiate genome engineering, featuring an illustration of two strands of a genome, the first top illustration showing the genome as three separate sections and the second lower illustration showing a D.N.A. break in the middle section so that there are now four sections to the lower illustration]
So, D.N.A. breaks initiate genome engineering. They initiate genome engineering because they initiate D.N.A. repair. And what genome engineers are doing is they are trying to essentially hijack the cell’s normal repair pathways. And in order to do that, you first have to trigger them. Okay? And so, you’ve got a double-stranded D.N.A. break. That initiates –
[slide animates on two more illustrations, one a duplicate of the D.N.A. with the break and an illustration beneath it wherein the break is rejoined and is labelled – End Joining]
– two main pathways. There are some variations on these themes, but there are two main ways that a cell can repair D.N.A. And so, this is obviously a really important job for a cell, right? So, things like U.V. light –
[Kate OConner-Giles, on-cam]
– can break D.N.A., X-rays, and we – cells have evolved to handle this. And so, one way that they do it is simply through just ligating the two broken ends back together, okay? This is called end joining or non-homologous end joining, and it is exactly what it sounds like. It’s a ligation of the two broken ends.
Now, the problem with this is that this is an imperfect process. And you can actually lose a base pair or several base pairs or gain a few at this junction. Okay. So, it’s an imprecise process.
[slide titled, D.N.A. breaks initiate genome engineering, with two new illustrations added to the right of the previous end joining illustration, these illustrations showing the initial illustration of the D.N.A. break on the top illustration, but now the bottom illustration shows a new edited D.N.A. strand in green with an arrow point to the lower illustration where this newly edited D.N.A. strand is placed in-between the middle ends of the D.N.A. break to create a homology-directed repair]
The other pathway is homology-directed. And what that means is that the broken end goes out and looks in the genome. It’s – its looking for the other allele. Alright. So, there’s a perfect copy somewhere else in the genome, if you’ve broken one chromosome. It’s going and looking for that, and then it uses, once it finds it, it uses that as a template to synthesize across the break and precisely restore the original D.N.A. Okay? So, any questions about that?
Okay, great.
So, these are the two pathways that the cell has available to it.
[new slide titled, How do you make targeted D.N.A. breaks, featuring two illustrations of a woman and a man which are made up of small green and blue multi-sized dots and whos have D.N.A. double-helixes in place of their legs]
And so, for a long time the really big challenge was HOW do you go in and make a precise break at ONE spot in a genome as huge as the human genome or even as big as the fruit fly genome? How do you do that? And there were ways to do that.
[Kate OConner-Giles, on-cam]
So, I – I guess I – I should back up a little bit and say the first thing you need is you need to know sequence. So, you need genome sequences. We’ve got that. As you’re all probably well aware, we have many, many, many organisms genome sequenced. So, you need the genome sequence, but you need more. You need a molecule – molecule that you can target to that sequence, okay?
And so, there were proteins that did this job. These proteins have actually been around for a couple decades.
[return to the D.N.A. breaks initiate genome engineering slide now featuring multicolored double-helixes floating above the initial D.N.A. break with an arrow pointing to that break]
So – so, CRISPR is not something that’s completely new in the sense that it allows us to do something we couldn’t do before. What is new is that it allows us to do something – it makes it feasible. It makes it reasonable to do it in – in a timely and an efficient manner. These proteins were very difficult to make. They didn’t target perfectly. And so, they were not really used –
[Kate OConner-Giles, on-cam]
– by many people even in a research setting, let alone in some of those other settings that we were talking about.
And so, many people, us included, were working very hard to try to find better ways to make a targeted break in D.N.A. And I’m not going to talk about this very much, but the couple things that our lab was working on, we were building on the work of Bill Engels and –
[slide titled, More efficient methods for targeting D.N.A., and featuring a headshot of researcher Bill Engels of U.W. Genetics and a photo of the transposons that he used in his work]
– colleagues from – from the ’70s and ’80s using transposons. So, the jumping genes discovered by Barbara McClintock in corn. To – when they jump, they actually break D.N.A. And so, we were working on using those as a tool for making targeted breaks in D.N.A. because in many model organisms, we have large collections of lines that have these transposable elements all over the place.
[the slide animates on two more headshots from researchers at U.W. Biochemistry, Mike Cox and Jill Wildonger, along with an illustration of their targeted D.N.A. break method]
We were also working in collaboration with Mike Cox and Jill Wildonger from the biochemistry department to try to bring a system from phage, which are viruses that infect bacteria, into other organisms. In our case, we were trying to bring this into fruit flies because that’s the organism we work with and the organism we desperately wanted to be able to just erase and change base pairs in.
And so, we were working on this –
[Kate OConnor-Giles, on-cam]
– and this is just sort of to give you an idea of kind of the setting. We were, you know, three of many, many, many people around the world who were trying to solve this problem when these papers came out in January of 2013. And it became instantly –
[slide titled, The CRISPR/Cas9 system, featuring the titles of three research papers regarding CRISPR technology – Multiplex Genome Engineering Using CRISPR/Cas Systems, R.N.A.-Guided Human Genome Engineering vis Cas9, and R.N.A.-programmed genome editing in human cells]
– clear that the problem was solved. And so, this – these papers showed the use of the CRISPR system to make targeted D.N.A. breaks. And it was immediately obvious to all of us who were working in the field that there was not going to be an easier way to do this. That this – this was so programmable that it – it – it was going to be difficult to top. It could be improved, but the – the – the basic concept was –
[new slide titled, CRISPR makes targeted D.N.A. breaks, featuring a D.N.A. strand shown as a horizonal black ladder with the targeted area colored green and labelled target]
– was what everyone had been dreaming of. And so, I’m just going to kind of walk through quickly how it works. So -so basically, what happens is you pick a 20-base pair target in the genome. Okay? So, pretty much any 20-base pairs to target.
[the slide pulls out the green target area leaving that area of the strand empty of ladder steps and those steps are now a new ladder underneath the target labelled g-R.N.A. which also has a smaller ladder tail attached to it at the end]
You make an R.N.A. that is complimentary to that target. So, it can bind that target through base pairing. Through simple base pairing. So, the logic is easy. The molecular biology is extremely easy. This is – will – will cost you, you know, 20 bucks and take you a day. And, you know, really a couple hours worth of work over that time. So – so, this is, you know, really easy molecular biology for any – any lab. Easy to program. Okay, so what that R.N.A. does –
[slide animates on a large grey circular area that encompasses half the remaining D.N.A. and half the target area as well as half of the g-R.N.A. strand including its tail and which is labelled Cas9]
– then is it binds this protein called Cas9, which is CRISPR-associated 9. Cas9 then is brought to that specific D.N.A. target –
[slide animates on two scissors, one at the top of the target area in the D.N.A. within the Cas9 and one at the bottom of the g-R.N.A. ladder within the Cas9]
– with the guide R.N.A. Cas9 is a nuclease, which is a protein that cuts D.N.A. Okay? Cleaves D.N.A. It has two nuclease domains, so it will cleave each strand of the D.N.A. And so that’s it. It’s as simple as that. You make an R.N.A. that is complimentary to the sequence in the genome that you want to target, you express this protein, Cas9, it will come along for the ride with the R.N.A. to that spot in the genome and make a double-stranded break. Okay?
So, pretty – so, I think you can see –
[slide returns to the initial ladder shaped D.N.A. sequence now with a break at the right-hand end of the green target area]
– you know, why – why were all so excited. This was – this was amazing.
[new slide titled, 1987 First I.D. of CRISPR sequences, featuring the title of the initial article regarding CRISPR sequences – Nucleotide Sequence of the iap Gene, Responsible for Alkaline Phosphatase Isozyme Conversion in Escherichia coli, and the Identification of the Gene Product – as well as an illustration of a C-G-T-A genome sequence]
And so, I just kind of want to walk through the history of this. You know, I think that some of the popular literature, in – in – and particularly in the media, sort of suggests it came out of the blue. And – and as all of you follow science, you know that’s not how science works. And so, we’re going to start our story in 1987.
So, what happened in 1987 was a group was just cloning –
[Kate OConner-Giles, on-cam]
– some gene in bacteria. They were working on their gene of interest. And they noticed next to their gene when they sequenced it that there was this weird sequence. It had these weird repeats and then with sequencing between that didn’t match anything that they knew. This was before any genomes were sequenced. So, that it didn’t match anything wasn’t particular surprising. But it was this real weird regularly repeating sequence. They mentioned in their paper, Oh, by the way, downstream of our gene is this weird sequence, we don’t know what it is. Over the years, by, say, 2000, there had been about 30 bacterial genomes sequenced, many of them here at Wisconsin.
[return to the First I.D. of CRISPR sequences slide]
And they started to recognize that these sequences were actually common in bacteria. And it turns out that 40% of bacteria have these CRISPR sequences, which stands for Clustered Regularly Interspersed, uh –
[laughter]
– Palindrome – Palindromic Spacer, something. Sorry ah, Short Palindromic Repeats. I always get that part wrong. So, it’s something that I don’t even remember and never say, so you don’t have to know it. It’s basically clusters of repeated sequence is what CRISPR means, okay?
Okay?
So, by 2002 –
[Kate OConner-Giles, on-cam]
– people who – who work on bacterium were interested in bacterial biology were working on this and interested in this really weird sequence. It must be something interesting, it’s conserved. They found that also conserved were these CRISPR associated proteins. So, we talked about Cas9. So CRISPR associated 9. A number of proteins were found to be always adjacent to these CRISPR repeats, and they encoded things, encoded enzymes that manipulate D.N.A.
So, enzymes, like nucleases that we just talked about, that cut D.N.A., enzymes that unwind D.N.A., things like that. So, this was starting to give them some hints.
In 2005, multiple groups reported that those sequences in between the regularly repeating sequences were invaders, like viruses. So, they were sequences from viruses and other things that invade bacteria that they may want to defend against. And so, in 2006, Eu-Eugene Koonin’s group hypothesized that this was actually an adaptive immune system. So, this was something that certainly was thought to be specific to mammals, that you could actually encounter a pathogen, have some way of remembering it, and going back and fighting it rapidly the next time you encountered it.
And this was tested in 2007 in Madison.
[slide titled, 2007 CRISPR adaptive immunity, featuring the title of an academic paper – CRISPR Provides Acquired Resistance Against Viruses in Prokaryotes, and also three photos, one of researchers working in a U.W. lab, and two of Danesco products from the research]
So, this was a group working at Danesco, which is a yogurt company in – in Madison. And so – so – so, cheese plays a huge role in the importance of this. So, they made – they made yogurt and pizza cheese, and their cultures kept getting contaminated. So, they were very, very, very interested in, for their yogurt cultures, finding ways of protecting them from these viral infections. And so, they tested this hypothesis. And what they did was they just selected virus – selected bacteria that could withstand viral infections, the ones that survived, sequenced them, found out they had CRISPR sequences from those viruses. Then they challenged them with another virus, sequenced the ones that survived, and they had incorporated into their genomes sequences from those viruses. And so, they were able to prove and then also they were able to show that then the next time they were exposed to –
[Kate OConner-Giles, on-cam]
– those viruses that they were again immune to them.
And so, basically, over that 20 years microbiologists were able to figure out that –
[slide titled, 2007+ CRISPR adaptive immunity, featuring an illustration of the cycle of adaptive immunity as a circle – at the top of the circle is the first viral infection showing the virus invading the bacterial cell, secondly a new spacer is derived from the virus and integrated into the CRISPR – this is labelled Adaptation – this forms a new CRISPR R.N.A – labelled Production of CRISPR/R.N.A., next the CRISPR R.N.A. guides molecular machinery to target and destroy the viral genome – this is labelled Targeting – from a second viral infection]
– what happens is that the – when a virus invades – let’s see if I can do this with my pointer. Okay, a virus invades, the bacteria actually snips out a piece of its D.N.A., sticks it in between these regularly repeating sequences in its genome. When it encounters that same virus again, it actually transcribes those sequences, makes that guide R.N.A. that then is complimentary to the virus D.N.A. It leads a nuclease, or a complex of proteins including a nuclease, to the viral D.N.A. and cuts it up, killing the virus. So, pretty cool.
So, why did it –
[new slide titled, 2012 Adaptation for gene editing, featuring the titles of two academic papers – A Programmable Dual-R.N.A.-Guided D.N.A. Endonuclease in Adaptive Bacterial Immunity, and Cas9-crR.N.A. ribonucleoprotein complex mediates specific D.N.A. cleavage for adaptive immunity in bacteria]
– take five more years for people to think about using this to solve that problem that I just presented at the beginning of this talk? It wasn’t that it took them so long to think about it. It was that there are many different types of CRISPR systems in bacteria, and it took that long before one that was simple enough, that only required one protein and one or two R.N.A.s, was found. The others required large complexes of proteins that would have been prohibitive for getting those into other types of cells.
And so, these – these two groups, Jennifer Doudna and Emmanuelle Charpentier, and then the Siksnys group, again with Barrangou, who was one of the guys who worked in Madison at the yogurt company and was one of the first to figure out its function in bacteria, what they showed was that you could use this in a test tube. So, in a test tube, if you put in the protein and the guiding R.N.A., the Cas9 protein and the guiding R.N.A., you could cut D.N.A. at will. So, you could program it to cut D.N.A. at specific sequences. So, this was really exciting –
[new slide titled, The simplified CRISPR-Cas9 system, featuring again a D.N.A. strand indicated by a sideways black ladder along with a green part of the ladder labelled target and now to the right of the target a small purple part of the ladder labelled PAM]
– and I just want to tell you how their system works exactly. So, I have to add one component to the story that I told you, which is this thing called the P.A.M., which is a protospacer adjacent motif. And what that is, it’s very important because if you think about it, I just told you that the bacteria insert the viral sequences into their own D.N.A., right? They have to have some way –
[Kate OConner-Giles, on-cam]
– of telling self from invader, and they can’t go around cutting up their own D.N.A. This is it. They always – every bacterial species will cut next to a specific sequence. So, when it snips out a piece of sequence, it only snips out sequences adjacent to a specific sequence. In the case of streptococcus pyogenes, which is the – the one that most genome engineers use, that sequence is N-G-G.
So, it’s not particularly limiting, but, basically, it means that as genome engineers we have to target sequences, we can target any 20-base pair sequence in the genome as long as it’s next to an N-G-G. If it’s not next to an N-G-G, the – the CRISPR/Cas9 system won’t cut it. Okay?
So, that’s a reason why –
[return to the simplified CRISPR-Cas9 system illustration, now with the target space emptied and the g-R.N.A. strand underneath the targeted section of the original D.N.A. strand]
-people are trying to gather these systems from many bacteria that have different P.A.M. sequences so that we can cut everywhere in the genome. And so, it’s not going to be a limitation. There are gazillions of these that have evolved. Okay, and so, I’ll just point out also that this guide R.N.A. that we use is actually a chimeric R.N.A. that was made by those two groups and discovered to work. There in the endogenous system in the bacteria, it’s actually multiple R.N.A.s. But so –
[slide animates on the Cas9 protein as a gray bubble as before and now it is noted that it also encompasses the P.A.M. part of the D.N.A. strand]
it’s been simplified –
[slide animates on the two scissors as before at the top of the target area and the bottom of the g-R.N.A. strand]
– to this system that I described –
[new slide titled, 2013 CRISPR engineered cell lines, featuring the titles of three academic papers regarding engineered cell lines – Multiplex Genome Engineering Using CRISPR/Cas Systems, R.N.A.-Guided Human Genome Engineering via Cas9, and R.N.A.-programmed genome editing in human cells]
– earlier. And so, using this approach that had worked in test tubes, three groups in January and February of 2013 – so, they showed that it worked in test tubes in August of 2012. By January of 2013, these groups had shown that it worked in human cell lines. Okay?
So, the – the potential –
[new slide titled, 2013 First CRISPR engineered organism, featuring a photo of a zebrafish and multiple yeast cultures in a petri dish]
– was – was immediately recognized. Within another month or so, other groups had gotten it to work in zebrafish and in yeast. So, some key model organisms.
[new slide titled, 2013 Germline transmission of CRISPR edits, featuring the headshots of the three lead U.W. researchers – Scott Gratz. Melissa Harrison, and Jill Wildonger, along with the title of their research paper – Genome Engineering of Drosophila with the CRISPR R.N.A.-Guided Cas9 Nuclease – as well as a photo of the flies that were used in the study]
We, in collaboration – so, this is Scott Gratz, a graduate student in my lab, and our collaborators, Melissa Harrison from biomolecular chemistry and Jill Wildonger from biochemistry – we got it to work in drosophila by May of 2013 and were able to demonstrate for the first time that it actually – this system could actually be used to edit the germline and to transmit changes to edited D-D.N.A. to the next generation.
And so, this kind of gives you an idea of the fact that it is actually pretty easy to get working. And –
[new slide titled, 2013+ CRISPR engineered organisms, featuring six photos of genetically engineered plant and animal species – a mouse, a plant, a pig, a monkey, a butterfly, and mushrooms]
– pretty much every organism in which people have tried to get this to work, it works. So, these are just a – a sampling of some of the many organisms in which CRISPR genome engineering has been successful. So, your model organisms, mice, followed shortly after flies, Arabidopsis –
[Kate OConner-Giles, on-cam]
– and other plants, pigs – there’s a – a – a – a big initiative to model some human diseases in pigs, which make better physiological models than mice in many cases – monkeys, butterflies, which many of you may know are studied in evolution and development, and then mushrooms just came out. How many of you saw the article about the mush – the CRISPR mushrooms? Yeah. So, just last week I think it was, or two weeks ago, the U.S.D.A. passed on regulating CRISPR engineered mushrooms. So, these mushrooms were engineered using CRISPR genome engineering to knock out the function of a gene that’s – thats involved in browning. And so, what this does is increases the shelf life, decreases the browning of the mushrooms and because the change involves no foreign D.N.A., the U.S.D.A. said it’s not a G.MO. And so that was – was pretty big news.
Okay.
[slide titled, CRISPR – the power of open science, featuring the logos of four CRISPR related organizations – CRISPR Resources, flyCRISPR, CRISPR Optimal Target Finder, and Cas9-triggered homologous recombination]
Alright, and so I just wanted to – also, I imagine that many of you who are following this are also reading about the patent wars. So, there are some major patent wars going on, and luckily, I work in fruit flies, so nobody cares. There’s no money to be made. So, I just wanted to give a – a shout out for the other side of this. So, the clinical applications certainly have, and agricultural and many others, have a great potential to make a lot of money. And so, this patent is important.
But even – but the groups involved – so, Feng Zhang’s group at M.I.T. and many other groups have been extremely open and generous in sharing with researchers, and that’s a major reason why this technology has spread so far and wide in labs.
And so, we, for example, have a website and some tools to help – that helped people get going in fruit flies. There’s similar things in worms. And so, I just kind of wanted to show the other side of this, that it actually has been a remarkably open process that’s involved incredibly –
[Kate OConner-Giles, on-cam]
– rapidly with lots of people sharing unpublished information to help pass on this really important lab technology for nonprofit research labs.
Okay. So, I told you that this solved that problem. The – the problem of how do you go in and make a break at a specific place in a complex genome?
[slide titled, Ongoing challenges to solve, featuring the previous illustrations of end joining and homology-directed repair]
That’s not to say –
[slide animates on a red circle around the illustration of the original D.N.A. break with the future challenge of increasing specificity and efficiency of D.N.A. cleavage]
– that there aren’t ongoing challenges. This system can and needs to be improved. So, we need to increase the specificity; people are working on this. This is happening. 20-base pairs isn’t – isnt that many and it actually can bind and cleave at not just the identical sequence, of course, if that occurs more than once in the genome, but at similar sequences in the genome. So, those are off target effects.
We also need to increase the efficiency. So, the – the efficiency is much, much higher than anything in the past, but it’s nowhere near 100%.
[slide animates on another oval red circle around the double arrows that lead to either the end joining or homology-directed repair options citing the challenge of controlling the cellular repair of D.N.A.]
And then here’s the big one. We can make the break, and then we pretty much can cross our fingers and try to bias things. But we don’t have control over which pathway the cell chooses to repair that break, okay? So, all we can do is try to bias it. And generally speaking, that means biasing it against end joining, which in many cells is the preferred pathway, towards homology-directed repair where the cell will repair not off of the homologue or a sister chromatid, depending on the point in the cell cycle, but off of an experimenter-provided piece of D.N.A. So, to make the edit that we want to happen. That is a huge challenge. And it’s not going to be solved any time soon. So, I think the first one is easier, and that one we’re going to get – get to faster.
So, I think that we don’t have to worry, for example, about designer babies next year or, you know, perhaps in any of our lifetimes. On top of the fact that we wouldn’t even know what D.N.A. to change, we can’t change the D.N.A. the way that we want to. We do not have that kind of control. So why, then, do we see – well, so let –
[new slide titled, A text editing analogy, featuring the sentence – CRISPR can be used to edit the Drosophila germlin – in a grey thought buble]
– let me actually back up a little. I wanted to – to – to give another analogy for – for – that – that path, the – the not being able to control the pathway. And this time I’m switching to text editing. So, how many of you text?
[laughter]
Not a lot.
[laughter]
Okay, half of you. So, it can be incredibly frustrating –
[the slide animates on the same sentence – CRISPR can be used to edit the Drosophila germlin – now with a cursor at the end of the word germlin]
– because your phone is competing with you to – to fix your mistakes. So, I, being a drosophila researcher who works on CRISPR, often, you know, will send texts along these lines that I’m sure my students are thrilled to get – CRISPR can be edited – can be used to edit the drosophila germlin.
[slide animates on the texts autocorrect option of gremlin instead of germline]
So, I left out the E. I, again, need to get my cursor in and go correct it to add an E, but my iPhone thinks I’m trying to say gremlin. And so, I have sent many texts about drosophila gremlins.
[laughter]
Because –
[the slide animates on the two options for the text – either gremlin or germline]
– my phone wins and I lose. And so, that’s essentially what we’re dealing with in the cell. The cell may be trying to write gremlin, and that’s not right. That’s not going to help the disease state. So, it’s – its a real – real challenge.
[new slide featuring three headlines about CRISPR – one from Science titled, The CRISPR Craze, one from The Independent titled, Exclusive: Jaw-dropping breakthrough hailed as landmark in fight against hereditary diseases as Crispr (sic) technique heralds genetic revolution, and one from Forbes titled, This Protein Could Change Biotech Forever]
Okay. So – so, why? Why, then, are we seeing headlines like this? You know, “the CRISPR craze.” “Jaw-dropping breakthrough.” “Could change biotech forever.”
[Kate OConner-Giles, on-cam]
First of all, there’s the prospect, but also there is the fact that under conditions where we can select for the events that occurred the way we wanted them to occur, this is paradigm shifting. This is changing the way we do things. This is a – a drawing that one of my – that one of the undergrads in the lab did for us. And this is really how we think of CRISPR. We can make any fly we want to make now. We’ve got a little machine. We put in a wild-type fly, and out comes the fly we want. It’s fabulous. We – we really have – have high efficiency, high success rate, but we can toss the flies that aren’t what we want. So that’s a very, very, very different situation than a clinical setting, of course.
So, the same is true for seeds, alright? And things like that where you could select for the change that’s the change that you want, toss anything that has an undesired change.
Okay. So, I thought I would briefly tell you, you know, what – what we’re using this for –
[slide titled, How do you build a brain, featuring an illustration of a brain that has several platforms around it and on the platforms are red 3D illustrated workers on the platforms pushing on the brain]
– in my lab. So, we’re interested in a big question – How do you build a brain? And –
[new slide titled, Building a brain that is both reliable and plastic, featuring a photo of a toddler playing with an iPad]
– more specifically, we’re really interested in understanding how you build a functional nervous system that can be both reliable, on the one hand – so, reliably controlled behavior – but also plastic change in response to the environment. And so, Im – I have a little video here that I hope is going to play. Oh, I’m afraid it’s not. Oh, it’s such a bummer. I’m going to describe it because it’s so cute. I – I – it won’t be as cute without seeing her. So, this is a kid who has an iPad, and so she’s learned how to use her iPad with her fingers. And then she goes to use a magazine –
[Kate OConner-Giles, on-cam]
– and she – the magazine is broken, and she gets really frustrated and she checks to see if it’s her finger that’s broken or what’s going wrong. But I really love it because it’s an example of, you know, over-learning, right? And so, you need – you need to be – your brain needs to be reliable, but it also needs to be modifiable. And so, this is a really complex problem.
The behavior, of course, is controlled by the brain. The brain –
[slide titled, Synapses are fundamental determinants of nervous system function and plasticity, featuring two illustrations, one of a brain on the far left, and next to it an illustration of a neural circuit]
– is organized into neural circuits. These neural circuits –
[slide animates on an illustration of a Neuron to the right of the Neural Circuit]
– their function is dependent upon the component neurons and their interconnections and their communication –
[slide animates on an illustration of a Synapse to the right of the illustration of the Neuron]
– at synapses. And so, my lab –
[slide animates off the illustrations of the brain, the neural circuit, and the neuron and then enlarges the illustration of the synapse so that it fills the entire slide]
– actually focuses on synapses. And so, we’re really interested in synapses both because these are the fundamental unit of communication in the brain, but also because they’re the site of plasticity. So, this is where the changes in brain function that underlie learning and memory occur. They occur at synaptic connections. It’s also becoming increasingly clear that aberrant synaptic function underlies many diseases. Many disease-states.
[Kate OConner-Giles, on-cam]
And so, we think that in order to understand the brain, you need to understand synapses. And, of course, I’m a geneticist, and underlying synapses are genes, right? And the – the genes that encode for proteins that function at synapses.
[slide titled, Identification of new regulators of synapse formation, function, and plasticity, featuring a photo of a gride made up of black, green, and red pixels which represent horizontally the genetic make-up at the embryo, larvae, pupae, and adult stages of fruit flies]
And so, what we’re doing with CRISPR and the reason we were so interested in being able to edit D.N.A. is we’re trying to take advantage of the genomic information that’s increasingly available –
[Kate OConner-Giles, on-cam]
– to identify candidate new genes that might be involved in synaptic function that are conserved from fruit flies all the way to humans. And then what we want to do once we identify good candidates is knock them out.
And so, we use a CRISPR based technique –
[slide titled, Identification of new regulators of synapse formation, function and plasticity, now featuring an illustration of gene deletions]
– to essentially just slice out the gene. We – we actually replace it with a red fluorescent protein that makes their eyes red fluorescent, the fruit flies’ eyes, because that allows us to follow the mutation. And it’s fun.
[slide animates on three photos, one labelled Morphology with a microscopic photo of a gene, one labelled Function that has two graphs on it that compares response times to stimulus, and one labelled Behavior, that features a photo of fruit flies in tubes in the lab]
And then what we do is we – we then screen these. So, we’ve made mutants in these genes we think are important and we test whether or not they have altered synapse morphology, altered synapse function, and ultimately altered behavior.
And in this way, we’re identifying interesting new genes.
[new slide titled, A structural gradient of active zones, featuring two illustrations – one of the original gene and showing g-R.N.A. of Green Florescent Protein (G.F.P.) being spliced into it, and a second illustration of the completed G.F.P.-tagged gene]
And then once we find new genes, we can use CRISPR to actually really get at their function so we can start to make more informative mutations than just getting rid of –
[Kate OConner-Giles, on-cam]
– the whole gene. We can change specific amino acids. We can knock out specific domains and really, you know, probe their function with mutations We can also, and this is one thing we commonly do, stick in a tag. And so, how many of you have heard of G.F.P.? Green fluorescent protein? Maybe seen green, fluorescent worms that you can buy at the grocery store? So, this is a jellyfish protein that biologists use a lot. And what we can now do is we can stick it into the genome and hook it up to our genes of interest so that when the cell makes our protein of interest, it will make a fluorescent version –
[return to the Structural gradient of active zones slide now with two illustrations at the bottom, one of G.F.P. and one of a Protein of Interest]
– of that protein. And we can follow it in cells. We can follow it in behaving animals. And so, we can see what these proteins are doing at synapses under different scenarios.
And so, CRISPR really is an incredibly valuable tool to us in the lab and has changed –
[return to the slide featuring eight photos/illustrations of the uses of CRISPR – pet, food production, biofuel, de-extinction, etc. from early in the talk]
– not just the way we do things but the things we can think about doing.
And so, with the – with the time remaining, though, I wanted to – to – to talk more about some of these other functions. Okay? So, specifically, I want to focus on –
[Kate OConner-Giles, on-cam]
– the idea of correcting disease-causing mutations in humans and targeting disease vectors. So, I think these are some really important potential applications of – of CRISPR technology.
And so, how many of you read about the CRISPR editing of a human embryo?
[slide titled, First CRISPR editing of human embryos, featuring the headline of a research article – CRISPR/Cas9-mediated gene editing in human tripronuclear zygotes – along with the definition of Beta thalassemia – inherited blood disorder in which the beta subunit of hemoglobin is absent or abnormal due to mutation of the HBB gene.]
Okay, very few. So, shocker.
So, they – so, this has been done. This was done in China. Human – human embryos have been edited using CRISPR technology. The embryos were nonviable. So, they – they were triploid. They had been – so – so, it was – in I.V.F., sometimes the egg will be fertilized by two sperm. And so, that’s not compatible with life, and that’s the embryos that they used to – to address potential ethical concerns.
And so, what they were trying to do was edit the gene that’s mutated in beta thalassemia, an inherited blood disorder. And so, I just –
[new slide titled, First CRISPR editing of human embryos, with the statement that they injected 86 embryos]
– wanted to kind of walk through what happened. So, they injected 86 embryos.
[slide animates on a downward arrow from the first injection statement to indicate that they sequenced 54 of the 71 embryos that survived 48 hours]
Of those, 71 survived 48 hours. So, putting the CRISPR/Cas9 components, the guide R.N.A., and the protein in wasn’t too toxic.
[slide animates another downward arrow indicating that of the 54 embryos that were sequenced, 28 were edited]
They sequenced 54 of those. Of those 54, half were edited. So, half there was no evidence of anything having happened, okay? I’ll point out that we can’t tell the difference between nothing happening and the cell correcting it properly, okay?
[slide animates on the left-hand side a downward arrow indicating that of the 28 edited, 17 had indels due to flawed end-joining repair, and featuring the two illustrations of end joining]
So, you know, correcting it right back to what it was before it was cut. Okay, so of those 28, 17 had those indels, which are the insertions or deletions that I talked about that can occur when you use the end joining pathway. This sloppy, error prone I should say, pathway. And so – so, they were potentially worse than they had started out.
[slide animates on another down arrow to indicate that of the 28 edited embryos, 7 used the highly similar HBD gene as a repair template]
Seven use the homology-directed repair pathway, but instead of repairing off of the corrected template – D.N.A. template that was provided by the experimenters, repaired off of a very similar gene in the genome, okay? And so, you ended up with the wrong gene.
[slide animates on another downward arrow to the right indicating that of the 28 edited embryos, 4 were repaired with the donor template, and featuring the two illustrations of homology-directed repair]
And then, in four cases, repair did occur with the donor template. So, we’re at four out of 86. So – so, not – not great odds for a clinical application.
[new slide titled, First CRISPR editing of human embryos (cont.), and featuring the statement that they observed significant off-target cleavage which is shown by three illustrations of three genome sequences with the target sequence on the top line, the second line showing the off-target site on the TTR gene in red, and the third line showing the off-target site of the C1QC gene also in red]
And I – I should say – so – so – so, some ethicists have –
[Kate OConner-Giles, on-cam]
– come out and said that, you know, that, This is ridiculous. This is just playing with human embryos. Everything that they saw, we see in the lab. Everyone has seen in every organism they work in. This is not new information. This is not unexpected. This is not a surprise that this is what happened. This is what happens when you do CRISPR.
They – other things that happen when you do CRISPR is off-target cleavage So, I told you that the guide R.N.A. can bind similar but not identical sequences. That happened in this case. So, even in those four where they got the repair that they wanted at the site they were targeting, they had off-target cleavage.
They also observed a lot of mosaicism.
[return to the First CRISPR editing of human embryos now with the statement of observed pervasive mosaicism, and featuring five lines of the gene sequences with the target sequence at the top followed by four examples of mosaicism in the gene sequence]
So, the two alleles would repair differently in a cell. Different cells would repair differently. So, they didn’t get whole organisms where everything had happened properly in all of the cells.
Okay. So, again, designer babies are not eminent.
[laughter]
[new slide titled, National and international initiatives to create ethical guidelines for genome editing, featuring three photos, one of a screenshot of the National Academies of Sciences, Engineering, and Medicine with an ad for an International Summit on Human Gene Editing, one a photo of U.W. law professor Alta Charo above an article titled, A prudent path forward for genomic engineering and germline gene modification, and one of researcher Jennifer Doudna at her desk]
But this certainly does have people thinking more about the – the prospect. And so, I just wanted to mention that there are lots of things happening to try to think about the ethics of using CRISPR/Cas9, or any other genome editing technology for that matter, to edit human embryos. Or – or humans. So – so, it doesn’t necessarily have to be embryos, it could be somatic cells in a – in a mature human.
And so, these are just some of the initiatives, and I wanted to highlight that our – our very own Alta Charo, is – who is a bioethicist in the U.W. Law School, is very actively involved in this. And they held a town hall a few weeks ago that was really interesting on the ethics of – of CRISPR editing. So, you might keep your eye open and see if they do that again. But very informative.
[new slide titled, Zika virus, and featuring four photos of infants (with their parents) who have been infected by the Zika virus and ended up with microcephaly – a birth defect]
Okay. So, I’m going switch topics and – and try to hit one more application before our time is up, and that’s the Zika virus. And so, as many of you know, this is a devastating disorder.
[slide animates on a map of North and South America and indicating in red the countries that have been affected by the Zika virus along with a list of the countries affected]
It’s currently affecting people in South and Central America. Pregnant women who are infected can give – give birth to children with microcephaly.
[slide animates on the photo of the mosquito on an arm from a previous slide]
And it is carried by the mosquito, Aedes aegypti.
[new slide titled, Gene drives spread in populations, featuring an illustration of how CRISPR could be used to eliminate Zika – on the top of the illustration are two mosquitos one wild and one CRISPR and showing that if they mate they would end up with two CRISPR offspring who would mate with two more wild-type mosquitos on the next line, and then their CRISPR offspring would mate with two more wild-type mosquitoes until on the bottom line all that is left is CRISPR mosquitos and no disease-bearing mosquitos]
So, what people want to do is something called a gene drive. Have any of you heard of a gene drive? Okay. I had not heard of a gene drive until about a year ago. It’s a very interesting – think of a selfish gene. So, it is a way of making sure that one allele is passed on to a 100% of progeny. And so, this – this deviating from normal inheritance patterns allows the new allele to spread rapidly through a population and take over.
Okay. So, how could you do –
[new slide titled, Gene drives spread in populations, featuring an illustration of a genome at the top with a section of g-R.N.A. about to be inserted in it followed by an illustration of the genome with two genes labelled HA1 and HA2 and with the Cas9 protein and the g-R.N.A. sandwiched in-between them]
– that with CRISPR? So, it’s kind of diabolical. And so, they did this in flies, and what they did was they put in a guide R.N.A. and they put in a donor template to target a gene. But what they did, that donor template actually had the D.N.A. to code for another guide R.N.A. and another Cas9 protein. And so, you replace a gene with the –
[Kate OConner-Giles, on-cam]
– molecules that will kill the other copy. Okay?
So, allele one you cut, Cas9 comes and cuts –
[return to the previous Gene drives slide]
– homology-directed repair occurs, and you incorporate the coding sequence for Cas9 and the guide R.N.A. targeting that gene into where that gene used to be.
[slide animates on another illustration showing the top genome having been cut by the Cas9/g-R.N.A. and beneath the cut is the HA1-Cas9-g-R.N.A.-HA2 splice]
So –
[slide animates an illustration of the new gene with the Cas9/g-R.N.A. directed repair in-between HA1 and HA2]
– homology-directed repair occurs, and that has now the – the replaced the gene. So then, what happens to –
[slide animates on the second allele showing that allele being spliced and converted to the same Cas9/g-R.N.A. gene as the previous allele without needing outside input from an experimenter]
– your second allele? Well, that first allele, the Cas9 is expressed, the guide R.N.A. is expressed, and it targets the second allele and converts it to itself. This time instead of using an experimenter – an experimenter-supplied D.N.A. template for repair, it uses the homologue. And it incorporates the Cas9 and the guide R.N.A. into its – its gene locus.
[the slide animates on an illustration of the homology-directed repair that happens naturally now in the original subjects offspring]
And so, you end up homozygous –
[slide animates on two identical genes side-by-side both now possessing the Cas9/g-R.N.A gene edit]
– for this. You pass on one copy to your offspring, they convert the other copy, and they are guaranteed to then pass it on to their offspring. And so, it rapidly spreads through the population.
This could be something that makes the animals die young. It could make them not mate. It could make them not able to be vectors for a particular virus. It could make them all male. You could do all sorts of things.
[new slide titled, Gene drives, featuring the title of an academic article – Highly efficient Cas9-mediated gene drive for population modification of the malaria vector mosquito Anopheles stephensi]
And so, this was done in fruit flies, and it’s now just last month been published that this was done in mosquitoes. And so, this has raised a lot of concerns –
[the slide animates on the article headline – Safeguarding gene drive experiments in the laboratory]
– about what the potential implications of such a – such a gene drive could be on the environment.
And so, many of us –
[Kate OConner-Giles, on-cam]
– who work with CRISPR/Cas9 genome engineering, in – in particular in flying insects where there’s a – a – a reasonable risk of escape from a lab, got together and wrote a policy statement. And you’ll note that this includes not just those of us who are – are – are – doing – using CRISPR/Cas9 for other things, but the authors who were using – who were creating these gene drives all agreed on safeties that needed to be in place in order to use these technologies that could potentially create a gene drive in the lab. And I’ll point out that nearly everything everyone’s doing in the lab could not possibly create a gene drive. So, that – its – it takes a particular type of experiment to – to cause that.
And so – so, this is what’s happening on the lab front, but it’s – its really – it’ll be interesting to see. I read a survey where the – the numbers were very, very high in terms of public support for wiping out Aedes aegypti if we can do it. So, I – I – I don’t know what’s going to happen, but it’ll be interesting to see. And CRISPR certainly does make it more possible.
Aw. None of my movies are playing. So sad. So, this was a worm.
[slide featuring a photo of a florescent drosophila larva at the top and a list of all the members of her lab that she wanted to thank]
This was not a worm. It’s a drosophila larva, and it has calcium indicators at all of its synapses. So, you can see them light up as it moves. Sorry I screwed up my movies.
[slide animates on the photos of Melissa Harrison and Jill Wildonger]
But I would like to thank the members of my lab, the current members, past members, and some of the undergraduate researchers who have worked with us on the CRISPR/Cas9 development side of things, as well as our collaborators, Melissa Harrison and Jill Wildonger –
[slide animates on the logos for the National Institute of Neurological Disorders and Stroke, The McKnight Foundation, and the University of Wisconsin-Madison]
– and our funding sources.
Okay, thank you very much.
[applause]
Search University Place Episodes
Related Stories from PBS Wisconsin's Blog

Donate to sign up. Activate and sign in to Passport. It's that easy to help PBS Wisconsin serve your community through media that educates, inspires, and entertains.
Make your membership gift today
Only for new users: Activate Passport using your code or email address
Already a member?
Look up my account
Need some help? Go to FAQ or visit PBS Passport Help
Need help accessing PBS Wisconsin anywhere?
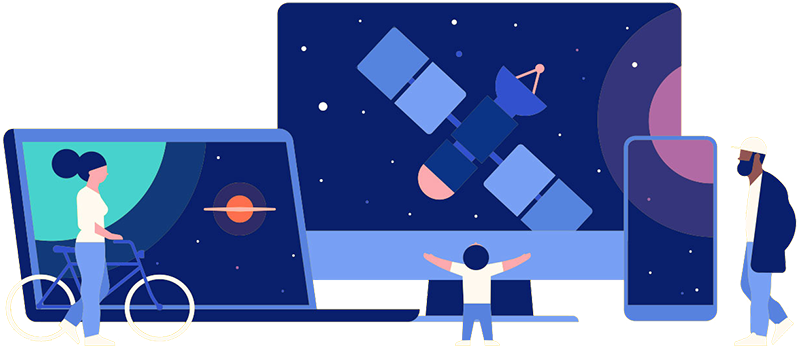
Online Access | Platform & Device Access | Cable or Satellite Access | Over-The-Air Access
Visit Access Guide
Need help accessing PBS Wisconsin anywhere?
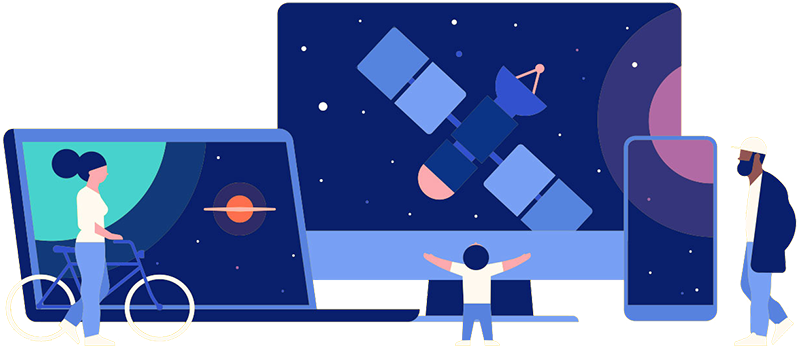
Visit Our
Live TV Access Guide
Online AccessPlatform & Device Access
Cable or Satellite Access
Over-The-Air Access
Visit Access Guide
Follow Us