– Jim Lattis: Well, good evening. Thanks for coming to Space Place tonight. It’s the second Tuesday of the month, and so we have a guest speaker tonight. And I’m very pleased to introduce Dr. Siyao Xu who comes to us from the University of Peking in Beijing, China, where she got her PhD in astrophysics. And is here, we’re lucky to have her here at the university as a prestigious Hubble Fellow, a NASA-funded fellowship. So she’s here to talk to us tonight about some of her research on astrophysical turbulence. So help me welcome Siyao. [audience applauding]
[Siyao murmurs] Yeah, it’s good.
– So this is a very beautiful starry night.
When we look up at stars through atmosphere, we see stars twinkle. This is similar to the city lights we see from a distance. When you look at a city lights from a distance, the city lights also twinkle. This twinkling of city lights and stars is caused by turbulence in atmosphere. Today, we are going to talk about turbulence in astrophysics. Turbulence is very common in our daily life. It exists in all types of fluid flows: in air, in water, in coffee, in smoke. And turbulence exists in contrast to laminar smooth flows. One exception is our, the blood flow in our body. Most time, blood also flows smoothy, linearly, but when we go to hospital to have a blood pressure measurement, then turbulence is generated in our vessels when the blood flow is partially blocked by the inflated cuff.
And this generation of turbulence produces sounds. We use sounds to measure blood pressure. This generation of turbulence in our vessels is very similar to turbulence generated in river, when the river is blocked by rocks. These rocks force the water flow faster through the very narrow space, then turbulence occurs. In this particular case of blood pressure measurement, turbulence is very helpful. But turbulence can also be dangerous. We frequently hear turbulence during a flight. For a nervous flyer like me, the bad news is that there will be more and more turbulence in the coming years because of global warming. But good news is that there’s also a forecast for turbulence that you can always check it out to avoid this possible turbulent regions before your flights. Turbulence is also very common in art.
This complex vortex features of turbulence have been captured by artists and leaving us these captivating paintings. To see how important turbulence is in art, let’s take this well-known Starry Night example. And we see from this painting, stars and clouds, they appear in turbulent swirls. This is very romantic picture. Can you imagine that if there’s no turbulence? [audience chuckling] Would you still enjoy the Starry Night without turbulence? To understand what is turbulence, let’s try to make turbulence first. You can do this simple experiment at home. Just turn on your water tap, but just turn on a bit when the water flows slowly, the water flow is laminar, smooth. But then you turn it up a bit to increase the speed of the water flow. Then the water flow becomes rougher and more turbulent. So the key here is the speed of water flow.
The higher the speed is, more turbulent it is. Let’s do another experiment. Imagine that it is not water, but some thicker fluid, like oil, like honey. And then you can easily imagine that even with the same speed of flow, it is more difficult for the thicker fluid like honey to get turbulent. The key here is viscosity of the fluid. Viscosity is the thickness of the fluid. It is the resistance of the fluid to any type of motion. The more viscous the fluid is like honey, the more difficult for it to become turbulent. Another example, for example, the more viscous chocolate river, like in Charlie’s factory, I always wanted to go when I was a kid, the less turbulent it is compared to the less viscous water river. So you see that the key here is viscosity.
From these experiments, we know that to generate turbulence, we need first high velocity speed of the flow, and second, we need smaller viscosity. And these gave us a very important parameter of turbulence: Reynolds number. Reynolds number is defined as the ratio between the inertial force and viscous force. Inertial force acts to promote turbulent motions. And viscous force acts to resist turbulent motions. Inertial force is related to, we already know, the speed of flow. It is also related to the size of the flow. For example, in the case of a pipe flow, the size is given by the diameter of the pipe. And the viscous force, of course, is related to viscosity. To have a turbulent flow, we need to increase the flow speed and the length scale of the flow, and decrease the viscosity.
Then we would have a large Reynolds number. When the Reynolds number is much larger than one, we have the inertial force much larger than viscous force, which means the inertial force can overcome the viscous force to generate turbulence in the flow. When the Reynolds number is much smaller than one, which means the viscosity is very important, the viscous force is much larger than the inertial force. Then it’s very difficult for the flow to become turbulent. We have a laminar flow. So Reynolds number is very important parameter to determine, to predict whether a flow is in laminar state or in turbulent state. Okay, so already notice that there is this length scale involved in the Reynolds number. And you know for astrophysical fluids, because of the very large length scales involved, the Reynolds number for astrophysical fluids are usually huge. It can easily go beyond 10 to the 10 order of magnitude. So astrophysical fluids are frequently highly turbulent.
In fact, the turbulence is ubiquitous in universe. It exists in different types of astrophysical systems over different length scales. It appears in atmosphere of planets, in atmosphere of stars like our sun, in protoplanetary disk where our solar system was born, in molecular clouds where stars are born, in our galaxy, in medium between galaxies. And note that these different systems cover a broad range of length scales, from kilometer to au, au, which is the distance between the Earth to the Sun, is approximately 10 to the 8 kilometer; to parsec, to 10 parsec, kiloparsec, to hundreds of kiloparsec. And know that parsec is approximately equal to 10 to the 13 kilometer. So this really huge range of length scales where turbulence exists. Well, we know that turbulence is very difficult subject. It is believed as the last, the oldest unsolved problem in both physics and mathematics. I was at a conference on turbulence last week in New York, and people from astrophysics, plasma physics, biophysics talk about turbulence. But we couldn’t really reach agreement on a clear definition, “What is turbulence?” But still, during these years, we still make significant progress in studying turbulence.
Well, just about I, we can see that turbulence is a multi-scale phenomenon. A multi-scale phenomenon means that turbulent motions, they not just only exist on a single length scale. Turbulent motions exist on different length scales. Similar to ocean bays, we see these huge waves on large scales and smaller waves on smaller length scales. This feature has been summarized by Richardson in 1922 in his poem: Big whirls have little whirls that feed on their velocity, And little whirls have lesser whirls and so on to viscosity. [audience chuckling] We call this whirl, this swirling structure in a turbulent fluid as a turbulent eddy. So the largest turbulent eddy break into smaller turbulent eddies. And these smaller turbulent eddies in turn split into even smaller eddies, until the smallest eddies where viscosity becomes important. So in this turbulence system, the energy comes from the largest eddy. Turbulent energy is injected at the largest length scale and then is passed down to smaller and smaller turbulent eddies, until reaching the smallest eddies.
Each eddy has its own length scale, velocity, and energy. In terms of the Reynolds number that we mentioned, for the largest eddy, the Reynolds number is also largest, because of the largest eddy has the largest length scale and largest turbulent velocity. And then we go down to smaller and smaller turbulent eddies with a decrease of the length scale and decrease of velocity. You remember the definition for Reynolds number, the Reynolds number decreases. And when we reach the smallest eddies, the Reynolds number is approximately one, means the balance between the inertial force and viscous force. This is the smallest length scale where viscosity becomes important, and this important viscosity kills turbulence. It is also the scale where the turbulent energy is dissipated. This energy transfer from large scales to smaller, smaller length scales is called energy cascade of turbulence. The energy cascade of turbulence is very similar to the cascade of water. If you imagine these different rock steps as different length scales, then the water energy is injected at the largest length scale, then cascades down to smaller and smaller length scale until reaching the ground, the smallest length scale, where the water energy is dissipated.
As a result of this energy cascade of turbulence, there is a power law spectrum of turbulent energy. This power law spectrum of turbulent energy tells us how the turbulent energy is distributed across different length scales. There is a very interesting feature of this energy cascade of turbulence. That is, this energy cascade has a constant rate. The energy transferred from one turbulent eddy to next smaller turbulent eddy has a constant rate, independent of the length scale, independent of the eddy we talk about. And this constant energy transfer rate gives a very particular slope, 5/3, of the power law spectrum of turbulence. And this was first discovered by Kolmogorov, so we call this Kolmogorov power law spectrum of turbulent energy. Okay, so now besides Reynolds number, we have another important characteristic of turbulence, which is this Kolmogorov power law spectrum of turbulence. We can look at Starry Night again. If you say this shows turbulence, it should have also a power law spectrum.
Recently, scientists did analysis, spectral analysis of this turbulent patterns in Starry Night, and indeed they found a power law spectrum. Well, the spectral slope is not Kolmogorov, is it a base steeper than Kolmogorov. Well, that tells us the turbulence in Starry Night maybe is a different type of turbulence. Let’s look at reality. So we know that our atmosphere is in constant motion. The spectrum measurement of the turbulent motions in atmosphere shows a power law spectrum. It is Kolmogorov power law spectrum. The turbulence atmosphere is very important for mixing, mixing the water vapor, the dust particles, the protons in atmosphere, and affect their distribution in both horizontal direction and vertical direction. And we are talking about astrophysical turbulence, so let’s look at turbulence in our galaxy. This is a power law spectrum measured from the electron density fluctuations induced by turbulence in our galaxy.
This is a power law spectrum, Kolmogorov power law spectrum spanning over a broad range of length scales from thousands of kilometers up to hundreds of parsec. And you remember that one parsec is equal to approximately 10 to the 13 kilometer. So this is over 10 orders of magnitude in length scales. And we call this big power law in the sky. Shows that our galaxy is turbulent over a broad range of length scales. Okay, so now we have learned some basic knowledge about turbulence; we know what is Reynolds number, what is Kolmogorov power law spectrum of turbulent energy, and we also know that turbulence exist everywhere in universe across different length scales. So how important is turbulence in astrophysics? Let’s first look at turbulent mixing. Again, we start with experiment we do every day: mixing coffee with milk. Imagine that if there’s no turbulence. Then this mixing happens depending on the fusion of molecules.
And these small molecules, they move very slow. So for the coffee and milk molecules to become uniformly distributed, for the coffee to become well-mixed, the mixing time scale is a few minutes. Do we usually wait for a couple of minutes for a cup of coffee? No, what do we do? We stir the coffee. Why we stir the coffee? To generate turbulence. And this turbulence acts to accelerate the mixing process. We can have a well-mixed coffee within a few seconds. And this turbulence mixing also happens in astrophysical fluids. Okay, let’s look at more experiments. So on the left side, this is the coffee experiment we just mentioned. On the right side, this is experiment we did in computer.
We put a blob of passive scalar, like a blob of dye, in a stirred turbulent box. And then we observed the evolution of this blob. Okay, let me play the simulations. And you see that this blob gets stretched, folded by the background turbulence. And it’s further fragment and becomes smaller and smaller pieces. Eventually, it is dispersed in the background medium. So what turbulence does here, turbulence, in fact, enhanced the area between the blob and the background medium. So it increased the area of the interface between different medium. So turbulence creates more chances for the mixing to happen everywhere in a turbulent medium. That’s how turbulence can accelerate the mixing process.
So this happens both in the coffee and in our experiment in computer. But I also want you to take a more careful look between these two experiments to see whether there’s a difference between these two experiments. Do you notice that on the right side, these fragmented filamentary structures resulting from mixing, they tend to be aligned in some particular direction? In the horizontal direction? The secret recipe here is that, besides turbulence, I also put magnetic field. And the magnetic field direction, you can guess, it is in the horizontal direction. That is why these filamentary structures, they tend to be aligned with the magnetic field. Okay, so I said mixing also happens in astrophysical fluids. And we already know that our galaxy is turbulent. So we naturally expect that to see such filamentary structures as a result of turbulent mixing in our galaxy. High spatial resolution telescopes reveal that, indeed, such gas filaments appear everywhere in our galaxy. And if you ask that whether there these gas filaments are also aligned with magnetic field, they are.
By comparing these gas filaments and the magnetic field environment, scientists find that, indeed, these gas filaments are well-aligned with the magnetic field direction. And so a clever way to measure magnetic field is to use this gas filaments as tracers to study magnetic fields in our galaxy. Don’t you feel that these gas filaments look very familiar? We also see such filamentary clouds in our sky as a result of the mixing of the turbulence in atmosphere. Turbulent mixing is also very important in the protoplanetary disk where our solar system was born around 4. 5 billion years ago. In this disk of gas and dust, turbulent mixing plays important role in affecting the chemical evolution, meteor distribution, and planet formation. As a record of the early stage of the solar system formation, meteorites carry important information on the composition of the disk material at that stage. So that is affected by turbulent mixing. So by studying meteorites, we can get a better knowledge about a turbulent mixing at early stage of our solar system formation. Okay, so that is about turbulent mixing.
The second important process induced by turbulence I want to talk about is called turbulent dynamo. Well, dynamo is a process, it’s a generator of new form of energy at a cost of another form of energy. For turbulent dynamo, it is a generation of magnetic energy at the cost of turbulent kinetic energy. Before we go to more details about turbulent dynamo, let’s first look at our universe. The structures of universe we see today, galaxies, stars, they are in a well-organized network, which is called cosmic web. The cosmic web is formed by all the galaxies and also the web-like strands that connect these galaxies. And these cosmic web formed from, initially, very small-density perturbations at early universe; and then these density perturbations got amplified by gravity, and then eventually, they formed the galaxies and stars we see today. Along this cosmic structure formation, magnetic fields in universe have also been amplified. The primordial magnetic fields in universe was extremely weak. And during this cosmic evolution, magnetic fields have been amplified.
So this is a simulation showing the evolution of cosmic magnetic fields. You see the different colors, the white color shows relatively stronger magnetic fields in galaxies, in cluster of galaxies. And these purple-blue colors show weaker magnetic fields in the strands connecting galaxies. So our universe, besides it is turbulent, it is also magnetized. Magnetic fields exist on different length scales, everywhere in universe, in this large-scale structure, this cosmic web; and also exist in smaller scales in galaxies, in stars, in planets. Do you know what is the magnetic field strength of our human brain? I check that it as weak as the magnetic field in the cosmic web, about 10 to minus 8, 10 to minus 9 gauss. So this is the magnetic fields we see today. And I mentioned that the primordial magnetic fields were very weak, so our universe was not born magnetized. The question is how magnetic fields have been amplified from its primordial form to the magnetic fields we see today? It is believed that turbulence, which is also ubiquitous in universe, accounts for the growth and maintenance of cosmic magnetic fields. We already said that turbulence is everywhere in universe.
It exists in medium between galaxies, in the medium between stars, in the medium between planets across different length scales. Do you know how the turbulence is driven in different systems? Turbulence can be driven by a creation of gas from the surrounding medium to forming this cosmic web. And turbulence can also be driven by collisions of galaxies. And turbulence in our galaxy is driven by explosions that follow deaths of massive stars. And you know that explosion can usually drive a lot of turbulence. And this driven turbulence in different environments further amplify magnetic fields by a process called turbulent dynamo. Okay, so as evidence that turbulence amplifies magnetic field, we can measure the energy spectrum of magnetic field. You remember that turbulence has a particular power law spectrum, the Kolmogorov power law spectrum for turbulent energy. The magnetic energy measured in different medium across different length scales also have the same Kolmogorov power law spectrum. And this fact shows that it is a universal process that turbulence amplifies magnetic fields.
Now, let’s look at how this turbulent dynamo works to amplify magnetic field. Imagine there’s a turbulent medium. Let’s put there some magnetic fields. You remember the experiment I showed you early that we put a blob in a turbulence medium, and this blob gets stretched by the turbulent motions. When we I put this magnetic fields in a turbulent medium, it is similar to the blob getting stretched. Magnetic fields also get stretched. After magnetic field gets stretched by turbulence, the magnetic fields get intensified. The magnetic field strength get amplified. This is similar to stretching noodles, if you imagine the noodles as magnetic field lines. So this tells us that amplification of magnetic fields where turbulent stretching, this is the dynamo process.
Okay, let me show you more illustrations. If you imagine that this boy as a turbulent eddy. And he’s doing turbulent motion. The noodle in his hands is a magnetic field line. So his turbulent motion stretches magnetic field line, and magnetic fields get amplified. And we know that along the turbulent energy cascade, there are different turbulent eddies on different length scales. And all these turbulent eddies can do the stretching motions, can amplify magnetic field on different length scales. And we said this during the dynamo process, turbulence kinetic energy is converted to magnetic energy, accounting for the growth of magnetic field. So one can measure the magnetic energy spectra at different time during the dynamo process. And you can see that the amplitude of the magnetic energy spectra which shows the magnetic energy, it increases with time, showing the growth of magnetic energy.
Also, it is important to see that the amplified magnetic field, indeed, follows this 5/3 Kolmogorov power law spectrum, the same as turbulent energy spectrum. Just as what we see in different astrophysical medium. Okay, so now we know magnetic fields are amplified by turbulence, and magnetic fields are ubiquitous in universe. Magnetic fields are also very important, and in fact, many astrophysical phenomena wouldn’t appear without magnetic fields. This is one example. You know, there are many dust grains in our galaxy. In the presence of magnetic fields, these dust grains can change their orientation. They can spin to the direction perpendicular to magnetic field. Because of this dust alignment perpendicular to magnetic field, the light coming from behind the dust and also the light coming from the dust itself becomes polarized. Polarization of light means that the oscillation of light has a preferred direction.
Because of this polarization of light, we can measure magnetic field in our galaxy. Here I’m showing the maps of magnetic fields measured by using polarization. On the left side, this is a map of magnetic fields measured by using the polarization coming from the emission of energetic particles spiraling along magnetic fields. On the right side, this is a polarization, it’s a magnetic field measured using the polarization from the dust alignment we just showed. And you can see from these magnetic field measurements in our galaxy, as amplified by turbulence, the amplified magnetic fields also have a quite turbulent structure. And all these maps tell us the magnetic field component in the plane of sky, perpendicular to line of sight. There’s another important magnetic effect, that is the polarization direction of light can rotate when there’s magnetic field along the line sight. And this is called Faraday rotation. And using this Faraday rotation effect, we can also measure magnetic field of a different component along the line of sight. And this is the map of the magnetic fields along the line of sight direction of our galaxy.
Okay, so this is about turbulent dynamo, that turbulent energy goes to magnetic field. There is another process in astrophysics which is opposite to this process, that is the energy comes from magnetic field goes to turbulence. That is called magnetic reconnection. So in normal conditions, magnetic fields do not interact with each other. But in some scenarios, if magnetic field lines with different directions come close enough, instead of forming a magnetic knot, they break and reconnect. This is called magnetic reconnection. Let me play this again. Okay, so the magnetic fields with different directions come close and close, and then they break and reconnect. So during this magnetic reconnection, part of the magnetic energy goes to turbulence. And as we said, that it usually doesn’t happen, because it’s very difficult for two magnetic field lines to become so close to reconnect.
But as magnetic reconnection can drive turbulence, and the turbulence, in turn, can accelerate this reconnection process. This is simulation showing that, so initially there’s no turbulence. But as long as the magnetic reconnection starts, turbulence is driven, and this turbulence can accelerate reconnection. So remember the experiment we said how turbulence can accelerate mixing. So turbulent can accelerate reconnection by creating more chances to bring magnetic fields become close together to make this reconnection process happen everywhere in a turbulent medium. That’s how turbulence can accelerate reconnection, so similar to a turbulent mixing process. So in the presence of turbulence, magnetic reconnection becomes very fast. So turbulence enables a faster reconnection process. And remember that reconnection can release a lot of magnetic energy that is stored in magnetic field. So turbulence enables a faster release of magnetic energy.
So this turbulent reconnection happens, for example, in the most energetic explosion in our universe, which is called gamma-ray burst. Gamma-ray burst is a short, intense burst of gamma rays. And we know gamma rays are much powerful than the visible photons in this room. The energy released by a gamma-ray burst in one second is more than the total energy released by our sun in its entire 10 billion years life. So gamma-ray burst is very energetic explosion in our universe. And gamma-ray burst is also a huge reservoir of magnetic energy. So it is turbulence that make turbulent reconnection happen, can have a faster release of this, a large amount of magnetic energy, and that enables this gamma-ray burst. Magnetic reconnection also occurs near the Earth. Our Earth has a protective magnetic environment, which is called magnetosphere. So at the boundary between this magnetosphere and solar wind, so solar wind is the energetic particles coming from the Sun.
And the magnetosphere works to deflect these energetic particles to protect us, the Earth. So the boundary between the solar wind and magnetosphere, there is a turbulent region, which is called magnetosheath. And this highly-turbulent magnetosheath, there is reconnection, which is turbulent magnetic reconnection. So this is at the head of magnetosphere. If you go to the tail of magnetosphere, because of the magnetic field lines, they get compressed because of solar wind, so you see the magnetic field line, they get close enough to reconnect. Magnetic reconnection process, I said part of the energy can go to turbulence, and part of energy can also go to particles. It is like a slingshot. The energy released in the reconnection process can accelerate particles. And these accelerated particles at the reconnection at the tail of magnetosphere, they stream along the magnetic fields, and then eventually, they reach the Earth. And these energetic particles, they hate the atoms in our atmosphere and force the atoms to give off light.
And that produces the very beautiful aurora we see. So of course, besides aurora, this reconnection event, they disturb the telecommunication, affects navigation, it may even harm the power grid. Okay, so this reconnection near the Earth. Reconnection also happens on the surface of our sun. It is the heart of most explosions on the surface of our sun in the form of solar flares. Okay, so this is a video showing these explosions induced by magnetic reconnection on the surface of our sun. Which is very frequent; it happens every day as a short release of magnetic energy. It happens everywhere on the Sun. [soft, ethereal music] Well, you know that magnetic fields are invisible. But because of the energetic particles that spiral along the magnetic fields can emit radiation, and that is what we observe.
So by observing this radiation, we can see the existence of magnetic fields, as this looping, arching structures through the atmosphere of the Sun. [soft, ethereal music] Well, as we said, that the reconnection also accelerate particles. So along the solar flares, there is also particles accelerated, and they are expelled from the surface of the Sun. That we call it coronal mass ejections, or CMEs. [soft, ethereal music] So here you can see the clouds of material of the energetic particles which are accelerated because of the reconnection events on the surface of Sun. So these energetic particles now are ejected into space. [soft, ethereal music] And then these energetic particles, they propagate in space from the Sun to Earth. So it’s similar to the the smoke particles which diffuse in atmospheric turbulence. These energetic particles from the Sun, they also spread out in space, and they diffuse in the turbulence medium in space. It usually take one day to several days for these CME events to reach the Earth.
And the CME, after reaching the magnetosphere of Earth, they strike the magnetosphere and disturb the space around the Earth, and this is called geomagnetic storm. To better protect our satellites, our spacecrafts from this geomagnetic storm, we need a better understanding of the parted acceleration due to the turbulent reconnection on the surfaces of our sun, and also these propagational particles through the turbulent space medium. Okay, so these are different process induced by turbulence. We talk about turbulent mixing, turbulent dynamo, turbulent magnetic reconnection. We also briefly mentioned turbulent diffusion of particles. There’s also other effects induced by turbulence like turbulent scattering. Turbulent scattering happens when light propagates through a turbulent medium. It causes blurring and twinkling of astronomical objects. It happens in atmosphere, it also happens in our galaxy. But on the other hand, we can use turbulent scattering to study turbulence in different environments.
There’s another effect called turbulent heating. We know turbulent energy cascade from large to smaller, smaller length scales until reaching the smallest length scale where turbulent energy is dissipated due to viscosity. And this dissipated energy, turbulent energy, is converted to thermal energy of the system. That heats the system up. So turbulent heating is also important energy source in many astrophysical systems. So see this, all this fundamental process induced by turbulence have important astrophysical consequences. And in this process, we know that there is energy flow from large to small length scales because of the energy cascade of turbulence. There’s also energy exchange among turbulence, magnetic field, and particles, where turbulent dynamo, turbulent magnetic reconnection, and reconnection acceleration of particles, and turbulence heating. So you see that turbulence has this unifying power to connect diverse astrophysical phenomena; from the large-scale structure of universe’s cosmic web to smaller-scale galaxies. From this most energetic explosions in universe, gamma-ray burst, to smaller explosions that happen frequently on the surface of a star, like the solar flares of our sun.
So you may not remember everything I talk about here today, but I want to stress that the simple physics we see in the phenomena in our everyday life, like physics of turbulence, also works, also happens, and plays important role in every corner of universe. Okay, so thank you for your attention. [audience applauding] Again, we start with experiment we do every day:
Search University Place Episodes
Related Stories from PBS Wisconsin's Blog

Donate to sign up. Activate and sign in to Passport. It's that easy to help PBS Wisconsin serve your community through media that educates, inspires, and entertains.
Make your membership gift today
Only for new users: Activate Passport using your code or email address
Already a member?
Look up my account
Need some help? Go to FAQ or visit PBS Passport Help
Need help accessing PBS Wisconsin anywhere?
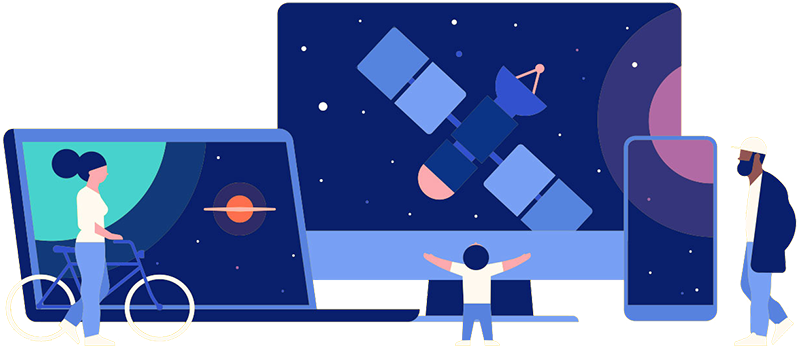
Online Access | Platform & Device Access | Cable or Satellite Access | Over-The-Air Access
Visit Access Guide
Need help accessing PBS Wisconsin anywhere?
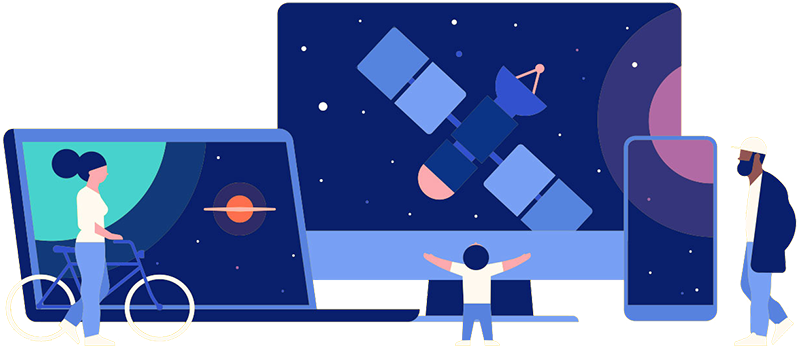
Visit Our
Live TV Access Guide
Online AccessPlatform & Device Access
Cable or Satellite Access
Over-The-Air Access
Visit Access Guide
Follow Us