[Tom Zinnen, Outreach Specialist, Biotechnology Center, University of Wisconsin-Madison]
Welcome everyone to Wednesday Nite at The Lab. I’m Tom Zinnen. I work here at the UW-Madison Biotechnology Center. I also work for UW-Extension and Cooperative Extension. And on behalf of those folks and our other co-organizers, Wisconsin Public Television, the Wisconsin Alumni Association, and the UW-Madison Science Alliance. Thanks again for coming to Wednesday Nite at the Lab. We do this every Wednesday night, 50 times a year.
Tonight, it’s my pleasure to welcome back to Wednesday Nite at the Lab David Gamm. He’s a professor of ophthalmology and visual sciences. He’s also with the McPherson Eye Institute and with the Waisman Institute, and with the Stem Cell and Regenerative Medicine Institute.
He was born in Colorado Springs, Colorado, went to high school in Grand Rapids, Michigan, then went and got his undergraduate degree at the University of Michigan in molecular biology and cell biology. Got a PhD at the University of Michigan, and then got a M.D. at the University of Michigan, and then he came over to the light side.
[laughter]
And came to UW-Madison to do his ophthalmological residency, and there are two Ls on ophthalmology, is that correct?
[David Gamm, Director, McPherson Eye Research Institute, University of Wisconsin-Madison]
Ophthalmology, correct.
[Tom Zinnen]
That’s I always went down on in the spelling bee. And he also did a fellowship with pediatric eye surgery.
That’s why I became a plant pathologist.
And now he’s going to talk to us tonight about making sense of stem cells, progress toward the treating of blinding disorders. Please welcome me in joining David Gamm back to Wednesday Nite at The Lab.
[applause]
[David Gamm]
Thanks Tom.
Well – well, thank you very much. I really enjoy the opportunity to come and talk to you. I – I talk to lay audiences quite – quite a bit, and this is a very sophisticated lay audience, so I’m trying to bridge my – my talks between the – the different levels that I try to hit. And I have a great deal of titles there. And I – I debated whether or not I wanted to put all of them up there, but I think it’s important that I do, because they all have a – play a big role in what I can do here at the university, and one of the – and – and why I am at this university. I came here for the – for a residency, as Tom mentioned, and stayed here because there were just way too many opportunities in Madison for me to leave. Then I’ve stayed here ever since, for reasons that I think would become obvious to you as my talk goes along.
So, I’m the Director of the McPherson Eye Research Institute, so we’re at one of the premier vision research institutes in – in the world. I’m also a part of the Department of Ophthalmology and Visual Sciences, which is one of the top clinical and research entities in vision science in the nation. And the Waisman Center is a wonderful place that looks at developmental disabilities. And so, my interest in blinding disorders fits in well with them. And then finally, the Stem Cell and Regenerative – Regenerative Medicine Center is – has been mentioned by many as being the Mecca of stem cell research in the world. So, Im really in a – in a – I’m privileged to be in a unique place on the planet to study what I – what I’m going to talk to you about today.
So, what does my lab do? So, my lab has a very clear mission –
[slide with the Gamm Lab Mission statement – To employ human pluripotent stem cell technology to develop therapies for the incurable blinding diseases of the retina.]
– and I try to bridge what I – what I do and see in the clinic with what I want to ultimately make changes in in terms of therapies for the future. And it – ultimately, what I’m interested in is using stem cell technology to try to build new treatments for blinding disorders that otherwise fall between the cracks.
[David Gamm]
Diseases that for which we don’t have any traditional therapies that can help patients.
And so, stem cells in general have – evoke a lot of questions in people’s minds, and they’re all very valid questions that deserve a lot of attention. And those include fields –
[slide with a graphic with the word Stem Cells in a circle in the middle with arrows pointing north to Science, east to Politics, south to Ethics and west to Medicine]
– of ethics, politics, as well as medicine and science. In the interest of time, I’m going to be concentrating on the medicine and science – the medical and scientific aspects of stem cell research, but that’s not at all to belittle the other ones. I think they are all very important sources of discussion that – that need to be addressed in public forums, as well as in scientific conferences.
[David Gamm]
And so, I’m happy to discuss those later on if there are specific questions.
Speaking of ethics, I need to disclose a couple of things right off the bat, so that you can decide whether or not what I’m telling you is the up and up, or whether I have some bias. I do have a patent. We patented the ability to create three dimensional human retinal structures in a dish.
[slide titled Disclosures stating that he has a Patent issued on 3-dimensional human retina structures derived from pluripotent stem cells with the logos for two companies based on this patent – Opsis therapeutics and Cellular Dynamics International]
And that has led to the establishment of a – a company, Opsis Therapeutics here in Madison that collaborates with another company, established by Jamie Thomson, and that’s Cellular Dynamics International. I do want you to be aware of that upfront.
[slide with an outline of Mr. Gamms talk]
So, what I’d like to go through today is basically two sections. The first is a lot of introduction. So, I’d like to give you a back – some background on cell biology in general, just enough so that I can then talk to you about the eye and the retina. What are its parts? What does it do? And then move into degenerative diseases of the retina; the specific types of diseases that I’m interested in. And then lead into a discussion of What is stem cell biology? And then that’ll form the basis for which we can go into a longer discussion about –
[David Gamm]
– how we’re trying to use stem cell technology to combat some of these disease – diseases. And then lastly, I debated whether I wanted to put this upfront, because I get the privilege of talking to you about a lot of his work, but there are tremendous number of people in my laboratory, collaborators here on campus and in other institutions, that play a tremendous role in advancing this technology. And so, while I appear to get the credit by talking about it, I want to make sure I have plenty of time to acknowledge the folks that are – that are doing a lot of the hard work.
So, to begin with, some cell basics. So, I apologize for folks in the – in the audience for which this is going to be really, really basic. But if we take a very stylized cell, which has a nucleus and a membrane, and all kinds of organelles within it that allows it to do its particular job. Some of those organelles are very basic –
[slide titled Cell basics with a graphic representation of a cell with a nucleus]
– and almost every cell in the body has them, and some of the specialty functions are – are specific to certain types of cells, and of course eye-receipting – light-receipting cells in the retina would be one of those very specialized cells that we’ll talk about it at length.
[slide adds a graphic representation of tissue as a collection of cells]
Cells combine together to make tissues, and multiple different types of cells are usually involved in – in production of tissues.
[slide adds a graphic representation of an organ – kidneys]
Those come together to make organs –
[slide adds a graphic representation of a system – the urinary system]
– and then organs are pieces together to make whole systems and, ultimately, organisms.
[new slide with a graphic representation of a healthy cell with a nucleus]
So, a healthy cell, if we’re all lucky, we – we have healthy cells throughout our life. But all too often –
[slide adds an arrow to a graphic representation of a diseased cell]
– we experience various types of diseases, whether it be an infection, whether it be a genetic disorder, whether it be an injury.
[slide adds a graphic representation of a therapeutic being applied to the diseased cell]
And in a lot of cases, if we’re lucky, there’s a therapeutic of some type, a drug, or a nutraceutical, or perhaps even something more advance like a gene therapy that can turn that diseased cell around and – and make it healthy again.
[new slide titled The dilemma of degenerative diseases with graphical representations of a healthy and a diseased cell]
The problem or the dilemma for degenerative diseases is that it’s kind of a point of no return. In these diseases, they become dysfunctional, but they’re still kind of alive and doing their thing. And this is when youre on the slope, the downward slope towards degeneration.
[slide adds an arrow down from the diseased cell to a graphical representation of a degenerated cell]
But ultimately, these cells break up, they die. They’re no long around, and there’s no way to put Humpty Dumpty back together again.
[slide adds an arrow from the degenerated cell to the healthy cell with an X through it]
And so, at – at that point, you’re kind of – where are you – where are you at? There’s no cell left for a drug to work on.
[David Gamm]
There’s no cell left to stick a new gene in, or to work on from a nutritional standpoint. So, in – in some tissues in the body, there’s an innate ability to regenerate cells. So, some cells naturally die. They go through a life cycle, and they need – need to be replenished periodically during your lifetime. So, they undergo to what we call natural cell death, not through the course of disease.
[new slide titled Cell regeneration with a graphic depiction of a healthy cell with and arrow to a dead cell in natural cell death and a smaller tissue specific stem cell underneath]
And in those – those tissues, there are resident stem cells that then can produce intermediate cells –
[slide adds an arrow from the stem cell to a graphic representation of an intermediate cell and then an arrow from the intermediate cell to the healthy cell]
– that then mature into and replace the healthy cells that have undergone natural cell death.
[slide titled Some cells and tissue regenerate with graphic representations of a bone and skin from a cellular perspective]
And tissues that do that are ones you’re probably familiar with, including blood. So, red and white blood cells –
[slide adds depictions of red and white blood cells to the graphic of the bone]
– are produced throughout the course of your life through bone marrow. So, there are bone marrow stem cells that you might here about, that people get transplanted with when they have, say, leukemia. And then the superficial portions of your skin are sloughing off, and then you make new skin cells over time.
So, those tissues have resident stem cells within them –
[David Gamm]
– that know when to kick in into cell division and to create more of the cell type that’s being continuously lost.
But many tissues in your body just don’t do that, so we – they have lost the ability to regenerate cells in some types of – of tissues in our bodies. And a primary one that we all think about is the central nervous system, so brain, spinal cord, and even retina –
[slide with a graphical depiction of the central nervous system showing brain, eyes and spinal cord]
– because the retina is part of the brain. It develops as part of the front part of the brain, and it’s made up of neurons like the brain. So, it’s a very specialized part of the brain, and it’s of course forward facing, and it’s a sensory organ, but it’s still brain. And like other parts of the central nervous system, it does not have a capacity to replace its cells once theyve been lost, either through disease or injury.
Other tissues such as heart muscle, insulin-secreting cells of the pancreas, as in diabetes, and cartilage also can’t replace themselves, but the level of need in terms of developing cell replacement therapies is different with these – these tissues.
[David Gamm]
Cartilage, there’s, you know, pretty good engineering devices that can be used to bypass the need for cartilage. Insulin-secreting cells, we have insulin, we have other drugs that can be used to maximize your own body’s ability to use the insulin that it produces. Heart muscle, if there’s just a little bit of – of heart muscle that dies, there are drugs that can be used to make the rest of the heart muscle work better, to try to overcome those diseases.
In the central nervous system, there’s almost no treatments like that, except perhaps Parkinson’s with L-dopa. But outside of that, when you’re thinking about Alzheimer’s, you’re thinking about degenerative diseases of the retina, or A.L.S., or Lou Gehrig’s disease, there’s really no place to turn.
So, prior to getting more into – into stem cell biology, I’d like to switch gears a little bit and concentrate on the retina. So, the rest of the talk now, I wanted to give you kind of a foundation where retina fits in terms of the landscape of tissues and application of stem cell technology. But from here on out, I’m going to concentrate on the retina. So, I’m going to give you an introduction now to basic retinal biology.
So, as an ophthalmologist, we like to dilate pupils, we like to look inside of eyes, and when we do that, this is what we see.
[slide with a photo of the inside of a normal eye]
So, this is a normal retina. The whitish, yellowish circle on towards the left is the optic nerve; this is where all of the nerve impulses that are collected in the retina from looking at objects gets collected and sent back to the brain. The red lines that you’re seeing are the – are the retinal blood vessels that feed the inner portions of the retina. And then the rest of it that looks kind of orange – the retina itself is really transparent like cellophane – the reason why it looks orange is that there’s a very vascular layer right behind it. And so, that red blood shining through the rest of the tissues makes it look orangish.
So that’s a healthy retina. And just like you only, like everyone tells you, you only use 10% of your brain.
[David Gamm]
For the most part, when us as humans, we use the central portion of our retina called the macula. And that’s just to the right of optic nerve in that kind of area where it looks like there are no blood vessels.
[return to the slide with the photo of the inside of an eye]
So that’s the very important part of our retina that we use to recognize faces, to read. It gives us our highest acuity vision and our color vision.
[David Gamm]
The rest, everything else around that is for peripheral vision. And there are some cones and some color sensation too, but that’s more for night vision and peripheral vision, but it affords us very poor acuity. So, the high acuity vision that we rely on so much is right there in the center.
And so, this would be then a normal visual field that someone might have with a healthy retina.
[slide with a photo of two kids dissolving to a photo of a single eye]
So, now let’s get – delve into this a little bit more. If we take that same eye and we cut it in half, and we look at it from the side –
[slide of the photo of the eye is cut in half and an arrow is added pointing to half of the pupil]
– we get this sort of schematic here.
[slide of schematic of a cross-section of an eye with all the pertinent parts labelled]
So, on the left side, that’s a – thats a cross section of your cornea. It’s clear. It’s part of the refractive portion of the eye that bends light. The oval to the right of that is then the lens, light passes through that, and then that gets refracted even more, and that’s actually a dynamic structure so it can refract light. Well, I’m starting to lose that ability, but when you’re young, you can refract light, and then adjust the focus of objects onto your retina.
And then on the very back, what – everything – everything that looks orange there is actually is – is a chamber filled with what’s called vitreous fluid, so that’s kind of a jelly that’s in there. And then along the back of the eye, like a wallpaper, is where the retina lies. It’s like film in the camera.
[slide of cross-section of eye animates a piece from the retinal and enlarges it]
And so, if you take a little piece of that retina and blow it up, and look at it on side – on the side –
[slide changes from the cross-section to a photo of a retina taken from the side]
– so now we’re looking at that piece of cellophane on its edge, and we’ve blown it up under a microscope, and we see that it’s really not just a boring piece of cellophane, but it’s actually a very elegant layer cake with multiple different cell layers, each of which does a very particular job. And it’s easiest to think about the retina as a simple brain that has essentially three neurons or three cells arranged in a circuit.
Deepest in the retina –
[a marking appears on the slide at the bottom of the photo indicating the photoreceptor cell – rods or cones]
– and this is why it’s transparent, but deepest in the retina, closest to the blood supply that makes the retina look orange, because it’s such a highly metabolic cell type, is our photoreceptor cells. These are the rods, which detect, you know, gray – gray vision – gray, white and black, and then cones, which are your blue, your red, and your green cones. They’re deepest within the retina in a layer called the outer nuclear layer.
[a marking appears underneath the photoreceptor cell indicating the location of the retinal pigment epithelium – labelled RPE]
And they exist right up next to and abut another layer of cells called the retinal pigment epithelium. And these are kind of like the Batman and Robin of vision. So, photoreceptors get all the credit because they’re the rods and the cones that detect light. But the retinal pigment epithelium is their – are their handmaidens. They do a lot of the work. They’re there to provide all the nutrients so the photoreceptors can stay healthy and – and active. And they also take away all the waste products that photoreceptors produce in large abundance and pull them away and – and deposit them into that blood supply behind it just so that they can get carried away. So, then you have photoreceptors and R.P.E. And the important thing to remember is that the R.P.E., because they’re so important for taking care of photoreceptors –
[David Gamm]
– if that layer dies, the photoreceptors aren’t long for the world. They’ll also go to, because they’re very needy.
Now, photoreceptors are – are then- then hooked up to another cell type the called type called a bipolar cell –
[return to the slide of the photo of the retina with animation adding markings of the bipolar cell and then the Ganglion cell above the photoreceptor cell]
– which in turn connect to another cell type called a ganglion cell, and you can see they exist in these different layers. And then Ganglion cells take – have their output processes or axons. Those are what coalesced to form the optic nerve to go back to the brain, which actually does the scene, right? Takes all that information, and then gives us a formed image.
So, you have a photoreceptor, a bipolar cell, and a Ganglion cell. And light passes all the way through this –
[a yellow arrow representing light moves from the top of the retinal photo to the bottom]
– so that’s why, again, it’s transparent, strikes a specialized structure at the very distal end of the photoreceptor cell, almost like a light-catching antenna called an outer segment.
[the yellow arrow turns to a yellow dot indicating light being caught by the outer segment]
That induces a series of – of chemical changes –
[yellow dot animates through all the areas of the retina to the optic nerve and brain]
– that ultimately lead to an electrical impulse that carries back through the optic nerve into the brain. Now, there’s processing that goes on in the retina, and there’s also processing that goes on in the brain that ultimately brings it all together and gives you your image. So, it’s much more complex than this, but we’ll – well keep it simple.
My laboratory is interested in that photoreceptor/R.P.E. cohort of cells.
[David Gamm]
So, while there are degenerative diseases that also affect Ganglion cells, so optic nerve disorders, glaucoma, my laboratory, while interested in that, doesn’t focus on that. So, I could certainly take questions later on about degenerative diseases affecting the optic nerve, but for the purposes of this talk, I’ll be concentrating on diseases that affect the photoreceptors or the R.P.E., which collectively are referred to as the outer retina.
This is what actual retinal pigment epithelium looks like.
[slide with a microscopic photo of retinal pigment epithelium]
So, if you were to peel back the retina, and just look at those handmaiden cells en face, so you’re looking at them straight down on them, they are actually pigmented, so they’re brownish black. And that helps absorb stray light, so you have less dazzling. So that – everything about these cells, there’s a purpose for it. And they look like little stop signs, and each one of those little stop signs, those little geometric figures that you see there, subserves multiple different photoreceptors.
[David Gamm]
So, they envelop them, and they provide the nutrients, pull away waste products. So, the photoreceptors sit on top of them, so they’d be going into the screen. Photoreceptors look a little prettier.
[slide with a microscopic photo of photoreceptors]
So, the reason it looks green or red is that we’re using special stains and antibodies that pick up on proteins that are specific for certain types of cells. In this case, the – the green color is due to a particular type of protein that’s only expressed in photoreceptors, and the red is only expressed in areas where there are synapsis occurring. And this picture, I got to give credit to Nico Cuenca, a friend of mine from Spain who is an outstanding histologist and makes just the most beautiful pictures from animal retinas. And this is actually a non-human primate retina.
And so, the lower part there, those little – little appendages, those little antennas that stick down, those are the outer segments. So, they’re stacked just elegantly so that you have multiple different – are called disks that are filled with proteins that detect light.
[David Gamm]
So, there’s essentially a perfect light catcher, and they’re arranged in such that if a photon of light comes through, there’s a good probability that one of these cells will catch it, and that will create the cascade of events that leads to an impulse going to the brain. So, that’s a very important part of these cells.
[slide with a stylized diagram of the parts of the retina]
And it’s showed here more stylized by those little lines you see going across. Those are the stack disks. Okay. And then below it is the R.P.E. cells, and you could see that they actually have appendages themselves, like little squid tentacles that go up and wrap around them. So, they really envelop and – and -and serve those photoreceptors.
[slide with a stylized diagram of the complete business of light detection in the eye]
And I’m not looking – ask-asking. There’s no quiz at the end of this, there’s no reason – I put this up here to actually read – actually for you to read, but the purpose is to show that this is a very complex cell. The – the business of detecting light is not easy at all. So, these cells have to have thousands of proteins –
[David Gamm]
– in the right place at the right time acting in concert. And so, when you start to think about trying to build these in a dish or trying to coerce cells to create them, it’s a pretty tall order. And so, even with the advent of stem cell technology back in the late ’90s, I can remember everybody saying, “Well, this is fine and good. We might be able to make a few little cells here and there, but we’re never going to be able to make something as complex as a photoreceptor cell.” And I hope to show you a little bit later on that I was – in many respects – this is – this is a recurring theme – wrong.
So, this is my attempt to make things kind of 3D. So, I had a little fun with this.
[slide with a 3D representation of the R.P.E. layer of the retina]
So, here we’re kind of looking at the same – just to try and give you another perspective on that, so here’s the R.P.E. layer, and you are kind of seeing it on – on tangent –
[slide adds 3D photo of the photoreceptor layer]
– and then on top of that then are the photoreceptor cells with their outer segments, their lighting catching antenna interacting with those R.P.E. cells. And so, if we kind of stack them up –
[multiple 3D photoreceptor photo layers are stacked on top of the R.P.E. layer]
– we ultimately get to this idea where there’s tens of millions of these photoreceptors subserved by all these photoreceptors, and they’re all working in – in concert.
[slide with a list of outer retinal degenerative diseases]
So, diseases of the outer retina. So, what happens in – in the diseases that I’m interested in my clinic and in my lab?
[retinitis pigmentosa appears in red box under the diseases heading]
So, the two broad categories of diseases that we look at are, one is retinitis pigmentosa. I’m a pediatric ophthalmologist, so this one is particularly near and dear to my heart, because it affects children. It affects children and adolescence, and then that’s when they first get it, and then it carries on into – into adulthood. It’s actually a group of disorders.
[David Gamm]
So, there’s over a hundred genes that can cause retinitis pigmentosa, but ultimately, they kind of all do the same thing, and that is lead to the death of particular types of cells in the outer retina. It’s relatively rare. As a total group, it has about two million people worldwide that are affected, but it’s the significant cause of blindness in that younger population –
[return to the diseases slide with pertinent information under retinitis pigmentosa]
– and it’s really devastating both to the patients, as well as to the families. You can imagine someone coming in, because their child just failed a vision test, and maybe they’re having a little trouble maneuvering around in the dark. And then you take a look inside their eye, you do a couple tests, and you realize they’re going to go blind in a few years, and you have to be the one to tell that to the parents. I don’t like that. Im about the second – I am number two in the room in terms of not wanting to hear or say that, and so that’s really what’s driven me in my laboratory, is to be able to say something a little bit more than “Here’s what you got, and I hope things workout well for you.” So, and again, almost no treatments are available. There are couple gene therapy trials out there right now that show some promise in terms of trying to gain a little bit of vision back. But for the most part, we don’t have anything that’s really very significant to offer these patients.
[age-related macular degeneration appears under the diseases heading in a green box]
Then the other group is on the other end of the spectrum, and that’s age-related macular degeneration. So, these – this is very common, so it’s a little bit different, in that instead of being relatively rare, it’s very common and growing, because our population is aging.
[pertinent information regarding age-related macular degeneration animates under the box]
There’s over 10 million people alone in the US that are affected, that will grow, that’ll probably double over the course of the next couple of decades. And it robs you of the central portion of your vision. And Ill – I’ll give you an example of that a little bit later on.
[David Gamm]
And unfortunately, although there are some therapies, there’s vitamins you can take to try to slow it down, if you got particular types of A.M.D., and there are injections you can get if you’ve got blood vessels that are contributing to your vision loss. But ultimately, there’s a death of cells, and once those cells are gone, there isn’t a drug or an injection that can bring them back, right now at least.
So, just to graphically kind of show you this. In retinitis pigmentosa, these are genetic disorders so these patients are born with a gene that ultimately fails them, and leads to death of the cells. Most of the time, that gene defect is expressed in the photoreceptors themselves, such that they cause primary loss –
[return to the 3D model of photoreceptors and R.P.E. with the photoreceptors disappearing from the model]
– of those photoreceptors over time, and that’s your slow inexorable loss of vision.
[return to a complete 3D model of the photoreceptors and R.P.E. with the R.P.E. layer disappearing from the model and then taking the photoreceptors with them]
In some cases, though, the gene is expressed in – the – the – the helper cells, the R.P.E. And as I mentioned, if those die, the photoreceptors then go, and then you get your vision loss.
[slide with a photo of the inside of an eye with retinitis pigmentosa]
And this is kind what it looks like. So, you could see it looks dramatically different than a normal retina. The optic nerve is there, it looks pale. The blood vessels are much thinner. You see all these kind of pigmentary change in the peripheral retina, which is a harbinger of loss of photoreceptors, and abnormal migration of the retinal pigment epithelium into – into the retina proper. And it affects generally, usually, the outer portions first, and then the central portions last –
[slide with a photo representing what a person with retinitis pigmentosa actually sees]
– such that kids do to pretty well, because they maintain their central vision, but they lose their peripheral vision and their night vision first. But ultimately, what happens in a lot of cases, unfortunately, is they eventually lose that central vision too –
[slide animates out the portion of the image that a R.P. patient sees resulting a black image representing blindness]
– and it could be devastating and lead to total blindness.
[David Gamm]
Not always, in fact, it’s kind of rare for you to be totally blind from R.P., but it certainly does happen.
Let’s contrast that to age-related macular degeneration, which doesnt – isn’t caused by any single gene, although there are risk factors for it.
[return to the 3D photoreceptor and R.P.E. model with the R.P.E. layer disappearing followed shortly by the photoreceptor layer disappearing]
But it primarily attacks the R.P.E. So, the R.P.E. is lost, and as I mentioned, because the R.P.E. is gone, the photoreceptors are gone too. And that’s if there’s no bleeding or abnormal blood vessels, then it’s called the dry type.
[return to the 3D photoreceptor and R.P.E. with the R.P.E. layer disappearing being replaced by blood vessels and eventual disappearance of the photoreceptor layer]
In the case of wet A.M.D., you have the R.P.E. that gets lost, and then those blood vessels that grow behind it, that are so important for serving the photoreceptors, abnormally grow up into the – next to the photoreceptors where they can break and bleed. And when that happens, it can lead to very rapid loss of vision, and loss of photoreceptors.
[slide with two photos of the inner eye, one with dry A.M.D. and one with wet A.M.D.]
And so, the dry type is shown there on the left. It looks just sort of like somebody took a blow torch and took out a lot of the retina. Those are – thats – that’s an area, like a cookie cutter, where all the photoreceptors are gone, and the R.P.E. is gone as well. On the right side, this is a patient that has something similar to the left, but also super imposed upon that has experienced a bleed.
[slide with a photo of an image of what a person with A.M.D. sees with no central vision and blurry peripheral vision]
And this is what patient with advanced A.M.D. would see. They wouldn’t go blind entirely, because their peripheral vision is unaffected, but they lose that central portion, and so you’re left with essentially very little ability to, essentially, for – to drive, to recognize faces, or to read, all these things that you really look forward to doing once you retire.
[slide with treatment strategies for R.P. and A.M.D.]
So, that brings us to some – there we are. If we have loss of cells, what – what are our choices?
[David Gamm]
Just theoretically thinking about this. If you’ve lost cells in the retina that are critical for detecting light, you essentially have two options at that point. You can either bypass the whole system. So, you say, “Okay, well, the photoreceptors are gone, so we have no natural ability to detect light, which means we’re going to not have vision in the area where the photoreceptors are lost. So, can we take some of the downstream cells, like the bipolar cells and Ganglion cells, and get them to detect light? That’s something called optogenetics. Or can we directly stimulate the Ganglion cells with a – with a chip? And that’s also available these days. Second Sight has a chip that will directly stimulate. And that has helped patients who are totally blind get flashes of light, detect movement, provide them some sort of – of vision. And that’s, while crude, something that has not been available decade or more ago.
And I bring this up to mention that there are other places you can turn, besides stem cells. What I think stem cells bring to the table is because it’s a natural replacement, the ceiling is a lot higher. And if we can get it right, we’re more likely to restore a more natural type of vision, as opposed to an artificial light on, light off. Okay?
So, my laboratory is interested in the idea of replacement.
[slide of graphic of treatment strategies with one arrow down to bypass and another arrow down to replace followed by a slide of the question of sources of spare parts]
And so, that brings up the next question, which is, okay, where are the spare parts going to come from? So, if you’re going to replace photoreceptors –
[David Gamm]
– or R.P.E. cells that are lost in disease, you gotta find some way – some place that you can get them. Living donors, so you can say, “What about a kidney? Can’t you take something from my other eye and put it into this eye?” Just like in the brain anything – when you mess with the eye, you’re going to blind somebody. So, you don’t have the ability to reach in and take your twin’s retina and try to replace something that’s been lost.
What about a deceased donor or a cadaver? Again, that’s been tried, and it just doesn’t hook up very well. So you have – if you take a mature retina that’s already been fully formed and try to pull it a part, they’re so fragile at that point that they tend to die, and they don’t hook back up again.
What about non-human? We used pig valves. And saw other animal parts sometimes we use for other tissues. That has not been directly tested in the eye, but the same thought would generally apply. That if you take something that’s fully formed, a fully formed part of the retina, just like a part of the brain, that those brain transplants, these transplants of fully formed neurons just don’t do a good job in these patients.
So, what about then lab manufactured? So, can we actually – can we make these cells in a dish –
[return to the sources of spare parts slide with the possible sources listed]
– usher them to an appropriate stage where we think they might be conducive to installing them and getting them to hook back up into a deceased retina, so that we can restore vision. And that was a great thought, and many people worked towards that, but there really was no source material for that.
[slide with a photo of the cover of Time magazine with Jamie Thomson on the cover]
Until in the late ’90s, this person Jamie Thomson, a collaborator of mine, and somebody that helped get me into the field, discovered a way to take a part of a five-day-old blastocyst, called the inner cell mass, and grow that in a dish. These were embryonic stem cells.
Everyone knew that they existed, because ultimately all of the tissues in our body –
[David Gamm]
– emanate from this mass of cells. But the ability to grow it in a dish in a laboratory had never been shown prior to Dr. Thomson’s work.
And embryonic stem cells are just one type of cells – of stem cells, and I want to take a brief detour just to mention that not all stem cells are created equal. So, people throw term stem cells around all the time, and there are different types of stem cells.
[slide with a graphic of an umbrella representing stem cells and underneath the umbrella tissue-restricted stem cells animates in]
So, there are what are called tissue-restricted stem cells.
[bone marrow stem cells animates in under the umbrella]
And those are like the bone marrow stem cells I mentioned earlier. These are resident stem cells in tissues that regenerate. They only have the capacity to make cells from which – from the tissue from which they come from. So, in other words, a bone marrow stem cells, you’ll say, “Well, I’ll get those bone marrows, stick them in my eye, you know. They’re stem cells, right?” Well, they can make blood, but they can’t make retina. Similar with skin stem cells and stem cells that line your gut. They can make cells that are normally found in those tissues, but they can’t make other cell types. And so, that’s a point of confusion that sometimes people who are trying to fool their customers try to use in order to get them to open up their billfolds and go towards a treatment that – that isn’t ready yet.
[pluripotent stem cells animates on under the stem cell umbrella graphic]
This is as opposed to pluripotent stem cells.
[slide with graphic representation of a pluripotent stem cell as a green circle with a black nucleus]
Pluripotent stem cells are an entirely different type of stem cell that is more primitive. And it has basically – it has two main properties. The first is that it has the ability to divide and make more of itself indefinitely.
[graphic of the pluripotent stem cell animates to reproduce two more identical cells]
And that’s important because it, theoretically at least, gives us an unlimited supply of cells with which we can perhaps make a lot of different types of tissues or – or – or cell types.
[graphic animates to show that pluripotent stem cells can be made to form different types of cells]
And then secondly, it has, if you treat it just right and push it down the right pathway, you might be able to get it to form multiple different cell types, so not just the cells of one particular tissue, but multiple tissues. And theoretically, perhaps even every cell type in the body. And that’s a very powerful thing –
[David Gamm]
– but it also makes it very difficult to work with. Because if you have essentially a clay-like cell that has the capacity to make almost any cell type in your body. That’s great, but the trouble is, you got to harness that someway, because you don’t want to just let it go willy-nilly, and make a hundred different cell types if you want, for instance, a photoreceptor. You have to find some way to get that to work in your favor, push it down a pathway, whereby at the end of the day you get the cells that you want and not a lot of contaminating cells that will just mess up your plans.
So, sources –
[slide with sources of pluripotent stem cells with a graphic representation of a blastocyst]
– as I mentioned before, there’s embryonic stem cells that are derived from blastocyst –
[slide animates on a microscopic photo of embryonic stem cells with arrows pointing towards the blastocysts]
– generally, from in vitro fertilization and eggs that are going to be discarded, and they’re shown here. So, each one of those circles there in the center in the square are thousands of individual embryonic stem cells. The other cells that are rounded are feeder cells. We generally don’t have to use those very much anymore, but those very bland-looking colonies of cells are what all the fuss is about.
[return to the graphic of the individual blastocyst with an arrow pointing to a graphic representation of a human being]
And they do indeed have the, at least, theoretical capacity to produce all the different cell types in a human, but it can’t make a human, but it can make all the different cell types.
[slide animates a different arrow from the human silhouette back to the blastocyst with the label induced pluripotent stem cell]
And then along around 2006, 2007, there – there came about another type of pluripotent stem cell called an induced pluripotent stem cell. And Dr. Thomson here at UW-Madison as well as Shinya Yamanaka in Japan –
[David Gamm]
– both almost concurrently figured out a way to take somatic cells, so cells from an adult human, and to genetically reprogram them back to a state where they’re very similar to, if not identical to, embryonic stem cells. So, because these are cells that were induced to become pluripotent, instead of being that way to begin with, they are called induced pluripotent stem cells. And this was a wonderful advancement, because it allowed us to make, essentially, stem cells, pluripotent stem cells from any human being on the face of the planet.
And here’s what they look like. So, they’re grown in the exact same way.
[return to the blastocyst/silhouette slide with a photo of induced pluripotent stem cells animated in]
They grow in these colonies that are relatively bland looking.
[slide with graphic representation of how induced pluripotent stem cells are created]
And ultimately, they can, at least theoretically, produce all the cell types of a human body.
So, but because they’re produced from adults, we can take, for instance, a patient that has a particular disease and through a skin or blood sample, reprogram those cells. So, these days, you can take a blood sample. Note, not much dissimilar from a sample you might send for a cholesterol check, and isolate certain cell types –
[David Gamm]
– genetically reprogram them back to this I.P.S. cell or stem cell state. And at that point, you have that particular patient’s genetic material encapsulated in their own stem cells. And if you have the ability then to push those, forward engineer those to, for instance, in our case, replacement retinal cells, then perhaps you could put that back into the patient.
[return to the slide of the creation of I.P.S cells with an added animation of cell therapy]
But along the way, you’re also going to have to probably correct if there’s a genetic defect. You want to correct that, otherwise you’re just going to have the same disease occurring again.
[animation of i.P.S. cells from a different patient added into the cell therapy graphic]
The other option would be to take another source of i.P.S. cells from a different individual who doesn’t have that particular disease, who perhaps is close in immune – is closely immune matched, and we’ll talk about that at the very end. Now, the last thing – something else that you could do with these i.P.S. cells that’s important to point out, but I won’t talk about, is something called disease modeling. In this case, you have these – these i.P.S. cells from the patient. And if you can create the cell types that are affected by that disease, you automatically now have an unlimited supply of that patient’s affected tissue in a dish. And it has the same genetic disease. It has all the other genetic makeup that that patient has. And so, you can study that disease, that patient’s disease in a dish, and then perhaps test drugs, test gene therapies, test more traditional approaches to therapy to see whether or not you can affect the course of that disease.
[David Gamm]
And by that – in that way, you can use i.P.S. cell technology to help the patient without having to put that cell necessarily back into the patient. So, I’m not going to go into that, but it’s a very powerful application of the technology that does not require transplantation, so keep that in mind. My lab, about half of my lab works on this type of disease modeling project.
But in both cases, like I said before, the trick is you have to make the cells. You gotta ultimately get these stem cells to create the cells that you need to study or to place back into the patient. So, how do we do that? In almost every case, we use development. So, developmental biology is our guide. So, stem cells are, during the course of their lifetime, normally, have to go down different pathways, are affected by different cues and factors, and that’s what tells them to go in a certain direction, become a certain cell or a certain tissue. And so, we try to recreate that environment and those steps in a dish to push them towards the cell type of interest.
In this case, this is a very early, just a couple week old, developing neural tube –
[slide with an illustration of retinal development showing parts of a neural tube]
– and the – and the future retina develops as a couple of budding balls on – on both sides of the front part of that future brain, and that’s called the optic vesicles. So, these kind of almost like a balloon being pulled out from the – from the neural tube. And it actually is a hallow vesicular structure.
[slide of retinal development from 28 to 44 days]
And this kind of more in 3D, what it looks like. You see it kind of folds up on itself and forms a hollow jawbreaker.
[slide of retinal development with illustration of a single eye]
And then over time, it folds in itself. It does it in some other rearrangements. And then ultimately, all those different layers of the neural retina are formed. The lens comes in. The cornea comes on over it. There’s a lot of coordinated activity that occurs to create ultimately the whole eye.
[slide showing all the steps a pluripotent stem cell needs to go in order to make it come out as a photoreceptor]
And between the formation of that optic – of – of that – of the front part of the brain to the formation of the optic vesicle, and ultimately the neural retina, there’s all kinds of forks in the road. And this is horribly simple, over simplified. But you can think about, you know, that you have to make decisions along the way. Am I going to become a part of the brain, or I’m going to be part of the gut? What, you know, what – what am going to become in a broad sense? And then you start narrowing yourself down as you move along in development.
And so, in some cases, there’s like natural clock inside the cells that tells them when it’s time to make a decision, but there are also other cues in the environment, and they could be diffusible factors or chemicals that are made by the embryo. They can be physical interactions where something pushing on another thing or touches another thing that it influences what it’s going to become ultimately. And so, it’s – its – its a tall order, right? We’re going to try and somehow recapitulate this in a dish.
[slide of building a human retina in a dish with a photo of human i.P.S. cells]
It turns out we’re pretty lucky, because a lot of it kind of does it on its own. And so, we do have to nudge it in certain critical times, but what we found is with just perhaps a few key cues or manipulations, then we could really get these cells to respond in a way that we – that we want to, whether that be Tim Kamp here trying to make cardiac myocytes for heart disease or Shusen Zhang trying to make dopaminergic neurons for Parkinson’s, or us trying to make photoreceptors for retinal diseases.
So, we’re going to go through just kind of on a high level what we do to get them to towards retina. Here’s, again, a colony. We have a couple of colonies of i.P.S. cells. We pick those up off the plate and let them grow in a three-dimensional structure.
[slide adds a photo of adding neural-promoting chemical cues to create a 3D structure]
As it turns out, anything that wants to be brain likes to grow on these free-floating structures. So, that’s by itself a step that helps us to get in the right direction, just a simple picking it up off of a plate so that it can grow by itself in a suspended aggregation. We also add certain cues to push towards an early brain.
[slide with a photo of the 3D neural structure in suspended aggregate]
And then over a period of time, we then plate them back down, so that they go from being a suspended aggregate to being stuck down onto a plate.
[slide adds a new photo of a cell that has been transferred from the aggregate back to a plate]
We switch the cues that we – that we provide them, again, trying to mimic development. And over time, we see them form these kind of very interesting radially oriented cellular colonies with almost like a little hole in the center.
[photo of the plated original cell transforms to a radial colony]
And we could take those off. We can actually pick those off –
[slide of a photo of the radial colonies with the holes circled]
– like this.
[slide of the radial cells as individual golden cheerios]
And so, when – when we pop them off, we leave behind all the cells that stay stuck, and we pick off these little circular structures which we call golden cheerios, and they’re actually vesicular little aggregates of cells, and they’re about perhaps the size of a coarse grain of salt, if you look at them in your hand. So, these are small. And so, we can pick them up, grow them on mass and flasks. And if you – if you look first at what’s left –
[slide with a photo of what is left in the dish after the golden cheerios have been popped off]
– so instead of looking at those golden cheerios, which is what we’ll spend time with for the rest of my talk, if you look at what’s left behind, so that’s the hole from where we picked off one of these vesicular type structures. And if we let it go over time and we treat it just right –
[slide with a photo of the R.P.E. that forms in the dish after the golden cheerios are out]
– we’ll find that, that skirt around those – where those golden cheerios used to be will become retinal pigmental epithelium.
[back to the slide of R.P.E. from earlier in the lecture]
And they will form the – the retinal pigment epithelial cells, not only in terms of what they look like, but they also can do all the same functions that we see in a normal eye. So, and we can make these, and this is now being done by many laboratories around the world. It’s also in clinical trials now, and I’ll talk about that at the end. But it’s really remarkable, we can make it, essentially, an unlimited supply of retinal pigment epithelium.
[David Gamm]
So, the spare part, at least for that support layer is not that hard to make, it turns out. And it kind of wants to do it. You have to almost make a mistake not to get this. So, that’s nice. Not the same for photoreceptors as I’ll show you.
So, let’s go back then to the little – little golden cheerios, the little vesicular structures that we picked up.
[slide with the photo of the golden cheerios enlarged]
So, these are hollow balls. These is under a microscope, so it looks kind a phase bright and golden on the outside but envision that they’re just hollow. They’re hollow balls. But are these really optic vesicles? Are these really those early structures that I showed you early – earlier in my talk that are budding off of the – the developing brain? And, you know, to be able to show that, I’ve gotta be able to follow these over time, and see what they make.
[slide changes to a photo of one of the golden cheerios isolated]
And so, let’s take a look at one of these.
[slide with photo of the cheerio with red and green indicating primitive retinal cells]
So, if I take a section of this, so if I take it and I cut it in half and look at it, and I stain it with something that turns primitive retinal cells, in this case, kind of a red or green color, you could see that it’s completely formed by these primitive retinal cells called progenitor cells. That’s important because it could be that there’s some retina in there and some brain. It could be a mixture of different things. But as it turns out, they grow separately, so that we can pick them apart from all the other different cell types. So, this then is the purification step that’s really important. We can pull it away from the retinal pigment epithelium, also if there’s any brain in the dish, or anything else that might be growing, we can pick it apart, so we can reduce the amount of contamination that might be there.
[slide with a photo of the cheerio after an additional week showing Ganglion cells occurring in purple alongside the red and green primitive retinal cells]
If we let it go another week or so, we start to see that it layers out. So those cells that make those progenitor cells, which have to make the entire retina, all those different layers, they move back and forth, and they start to make the cell types that are normally found in the retina. So, here in the purple are the Ganglion cells, so those output neurons. They’re born first, normally, when the human retina is being formed. And they’re born first in the dish as well, and they form a layer like you see there.
[slide of a photo of the cheerio after several weeks showing primitive photoreceptors in red and yellow]
Then a little bit later on, we see production of primitive photoreceptors. So, in red and yellow are particular fluorescent markers that home in on cells that have early characteristics of photoreceptors. So, these wouldn’t be able to detect light yet, but they’ve already decided that they want to become photoreceptors. The question is how close to being a mature photoreceptor will they ultimately become.
[slide with a photo of a cheerio at 200 days in a dish]
And so, it takes a long time. So, we have not found a way, very well at least –
[David Gamm]
– to be able to accelerate the process. So, one of the downfalls of this – from a development- from developmental biology standpoint, it’s great, because we love to be able to – to follow development in a dish. But from a cell manufacture standpoint, we haven’t been able to overcome the clock. So, these cells march along from immature to mature, but they take their own sweet time. And essentially, the amount of time it takes is almost identical to what it would take in a developing human embryo. So, it’s really kind of remarkable that the timing, that clock stays the same. But here at 200 days in a dish, so this is more than, you know, more than half a year, it’s gotten big. Not this big, but it’s gotten bigger. And you see on the edge, a lot of little hairs.
[return to the 200 day photo]
Perhaps you can see it. I hope it conveys well on TV as well. But there are these kind of little hair-like structures that cover the – the outer surface.
[slide of cheerio again at 200 days, showing primitive photoreceptors in green, cones in red and rods in purple]
And if we cut these again in half, and we look at mature markers now. So, now we’re looking at markers, fluorescent tags, that tells us whether you’re not just a photoreceptor, but are you a cone or are you a rod? In red here are the cones. In blue are the rods, and green is kind of a mixed marker, so it’s kind of both. And so, not only does it make cones and it makes rods, these are red/green cones, but they actually form the right layers. Cones are usually on the very outermost portion of the photoreceptor layer. Rods tend to be on the inner portion. So, we’re not doing this. We’re not moving things around. We’re not taping anything. It’s just doing it on its own. So, it kind of knows where it wants to be relative to other cells as they’re being born.
[a box appears in the slide showing an actual human retina with cones in red and rods in blue compared to the section of the cheerio]
And if you compare this now to, in this case, a human retina, so this is not grown in a dish, but an actual human retina, and overlaid here, you could see how close it is. So, the little red structures are a little bit longer than what we see in the dish, but the relationship is all there. And in some cases, it’s kind of hard to tell the difference between what’s grown in a dish and what we can see in – in a donor eye, for instance, from a cadaver.
[slide with a close up of the outer layer of the cheerio}
Okay, so our interest then was piqued because we see that they’re separating out like they should, their relationships are correct, they also make long processes that form synapses that I’m not showing you, but they do that as well. But what I was particularly interested in was these – these little hair-like structures –
[the outer layer of the cheerio is highlighted in a box with red hair-like structures and the question of light-catching outer segments]
– because is it possible that these could be early outer segments? So, this little antenna that detect light, because that’s really critical. If we put a cell into somebody’s eye and it can’t make an outer segment, then it’s essentially just maybe –
[David Gamm]
– a cell that survives and hooks up, but it has no ability to detect light. And this is an exceedingly specialized structure that only photoreceptors have. So, we kind of looked at that really carefully, using electron microscopy, and sure enough, that’s what they are.
[slide adds an electron microscope photo of the hair-like structures enlarged]
So, they don’t look great. We call them little pod people. But this is actually – this is what outer segments look like when they’re first born. They don’t start out long and with perfect stacked disks ready to catch light. They start out as these little pods which then elongate and grow. And we have older ones that do date 250 days up to 300 days, where they do get longer, and the disks starts to stack appropriately. But this little area here – if I can get it to work – is called the connecting cilium, a very specific structure that then ends in an outer segment. So, these are all – we – we were just amazed when we saw it. And again, we’re not doing too much. We’re providing it some cues, but for the most part, these calls are pre-programmed to go this route once you get them going in the right direction – which is wonderful because, ultimately, if we put them into a person’s eye, we’re not going to be there to squirt them with a bunch of different factors every three minutes to make sure that they do what – what they’re supposed to. To some degree, we’re going to have to set them on the right path, and they’re going to have to do the rest of it themselves. And it looks like that, in fact, can happen.
[slide with a photo of a dish cell detecting light by UW-Madisons Bikash Pattnaik]
So, if it has the right properties and the right morphology and structures to detect light, can it actually detect light? And for this, we collaborated with a wonderful electrophysiologist here on campus, Bikash Pattnaik, who’s in the Department of Pediatrics. And he could actually lower an electrode onto one of these cells, here shown in red. We got all the photoreceptors in this culture to glow red. And then we recorded from it –
[slide animates on graphs of the various recordings that the team did]
– to see whether or not it would respond to cues that a photoreceptor usually responds to. Now, we cheated a little bit here, because we bypass the need for light.
[David Gamm]
We instead gave it a chemical that’s one step beyond the detection of light, but it responded. It responded just like a normal photoreceptor cell. We could – we could mimic the light being on and the light being off, and it would turn on and turn off just like a photoreceptor cell normally does. And then another collaborator, Dr. Canto Soler from Johns Hopkins who’s in now at the University of Colorado. We collaborated with her; she took it a step further and actually looked light impulses with a little more mature cell and – and showed that they in fact can respond to light with an electrical impulse. And this is the first time that any photoreceptor had been shown to respond to light in a dish. Even if you take a real photoreceptor from an animal or a human, and take it out of its natural environment, put it in the dish, it will no longer respond to light. So, this was the first time that any photoreceptor from any source in a dish had shown the ability to respond to a photon of light.
So, we’re obviously very thrilled. But along the way we’re thinking, “Well, our ultimate goal – now, it’s wonderful that we can use these, like I said before, to model disease, so we can take, for instance, a patient that has retinitis pigmentosa from a genetic defect that affects the outer segment. And now we can grow those in a dish and try to see if we can find drugs or other therapies that might help that patient. But if we want to take that cell, once that cell is gone, there’s no way that the drug is going to help them. So, if we’re going to take the cells that we’re making and put them into that patient, we cant just – we have to move out of the laboratory at some point. We’ve got to find a way to take what we do in a dish and convert that to a method that’s suitable for use in humans. And that’s an exceedingly difficult thing to do, but we’re unique suited to do that here at the University of Wisconsin, because we have a biomanufacturing facility on campus. And in fact, it’s two floors down from my lab.
My lab is the sixth floor here on the Waisman Center.
[slide with a photo of the UW-Madison Waisman Center with two smaller photos – one of the Waisman Biomanufacturing logo and another of a scientist in the lab]
It’s on the far western portion of the campus, on the medical campus. And then the biomanufacturing center is here on the fourth floor. And it’s a world-renowned center for producing self-therapeutics, also gene therapies, and – and pharmacologic therapies as well. And I had the privilege throughout the course of my career here, as I’m developing these therapy – these cell types, to walk down –
[David Gamm]
– and talk to the people there and say, “Okay, well, how do I ultimately convert this to something I can help a patient with?” And so, and most people can work 20 years until they finally get to talk to somebody that has that kind of expertise, and then they find out they have to do everything all over again, because they made critical mistakes along the way, unbeknownst to them. Whereas, I’ve got people coming up and saying, “Don’t do that. That’s not a good way.”
[laughter]
“If you’ve got this choice, choice A and choice B, go B. A will never get into a human, B will.” And so, it saved me a lot of time, and I’m very grateful for that, one of many things I’m grateful for, to be a scientist here at the University of Wisconsin.
So, with the collaboration with that – that group at the Waisman Biomanufacturing Center, they were able to recapitulate our method using techniques that are compatible for human use. And so, you could see lots of these little optic vesicle structures.
[slide with a photo of clinical grade photoreceptors from human i.P.S. cells]
[slide animates to dyed photo of clinical grade photoreceptors in green, red and yellow]
These green, yellow and red dots are all photoreceptors that have been broken apart from these optic vesicle structures as they’re grown. So, we grow them up, and then we kind of piece them apart, so we can get kind of a – a – for lack of a better term – a little handful of photo receptors.
[slide asks the question of installation]
And so, it’s very – its possible now to be able to not only create the photoreceptors, but do them in such a manner –
[David Gamm]
– as to make it compatible with human use. That’s all cell production. And so, if you think of a Michigan analogy, a Detroit analogy, that’s making the spare parts. But ultimately, what about installation? And I’m bringing that up at the rear not because it’s less important. It’s actually as important, if not more important, and an even greater challenge.
It’s great that we’re at this position where we’re now thinking about how best to install the cells because we actually have the spare parts now, which 10 years ago we didn’t have, but it’s still a large hurdle to overcome. Taking a cell that’s brand new and putting it not just into another human’s eye, but a diseased eye. So, it’s an environment then that’s going to be slightly toxic. The cells have – have degenerated away. There can be scarring. There can be remodeling of other cell types within the retina. So, there’s a lot of factors that need to be considered when talking about putting the cells back into an eye and getting them to hook up and restore vision.
Thankfully, we’re ahead of the curve on this simply from the fact that the surgical tools and the surgical skills are pretty mainstream. So, when you think about trying to put in dopaminergic neurons in the middle of somebody’s brain, boy, cell replacement in a brain hasn’t been done very much. But the eye is very accessible and the surgical techniques and tools are all pretty mainstream. So, a lot of retinal surgeons, when dealing with retinal detachments and other types of diseases are pretty comfort – comfortable able getting underneath the retina and manipulating things.
[slide with an illustration of transplanting human stem cell-derived photoreceptors]
So, the approach is relatively quick, and the risk is pretty low. Plus, you’re dealing with two organs, right? So, you got two eyes, not one, so you kind of have one to play with maybe –
[laughter]
– especially if neither one of them work, right? And if there’s an untoward effect, like everyone worries about tumors, it’s an encapsulated organ, so you’re less likely to run into trouble. And as much we hate to do it, we can take that eyeball out. So, if anything, worst case scenario, you can’t take your brain out –
[David Gamm]
– but you can take your eye out. And then lastly, we have the tools to look at – at what’s going on in real time. We don’t have to put you in an M.R.I. machine or a P.E.T. scanner to see what the cells are doing. We just dilate you and take a look. We have all kinds of instruments that can give us 3D images of what’s going on underneath that transparent retina. So, it’s not behind a curtain, it’s right there in front of us, so we can follow what they’re doing from a functional standpoint, as well as an anatomical standpoint in real time. So, all of these things have led the eye to be really on the forefront for cell replacement therapies using stem cell technology.
Here’s an example –
[slide with a photo of an enlarged retina showing donor photoreceptors and a host retina]
– of where we took the donor human photoreceptor cells derived from i.P.S. cells are in red. This is actually in a rat that has a disease that causes all the photoreceptors to go away. And so, there are no photoreceptors in this rat. The blue and the green are the remaining layers of the rat that still are there. These are blind rats. And you can see how we can inject the cells and get them to at least reform a – a layer. It also forms connections and synapses with the – the host rat as well. We have to manipulate the immunology of the rat, because it’s a xenograft. It’s a human into a rat, so that rat tends to destroy it. So, we have make to sure the rats don’t have a proper immune system to do that. So, there’s some manipulations that have to occur, and a rat is not a human. So, it’s promising, but ultimately until we get into humans, we aren’t really going to know.
[slide with a photo of custom photoreceptor scaffolds]
But that approach is really just taking out that gemisch of photoreceptors and injecting that into the subretinal space, which is awfully crude, right? We’re – were making them. We’re really proud. They make photo – they make outer segments. But I showed you a way in the beginning that these photoreceptors aren’t just randomly interspersed in your retina. They’re tin soldiers. They have an up, they have a down –
[David Gamm]
– they have an interconnection between one another. They have a particular orientation that’s important for them to work correctly. So, if we’re just randomly squirting them into the subretinal space and hoping just to sort themselves out, yes, it’s shown some promise, but it’s awfully inefficient as you might suspect. So, we’re – were looking ahead and saying, “Okay, well, how can make a version 2.0? How can we work on version 2.0 while we’re still trying to push 1.0 along?” And one way to do that is to put them into prefabricated bioengineered scaffolds. And reason number 305 Im at the University of Wisconsin, we got a great bioengineering group, and they’re very collaborative, and they love this sort of stuff.
So, you walk to them, you say, “This is what I got. I got these cells, but they’re all kind of randomly dis-dispersed when we disassociate them out. Yes, we’d love to put in the whole, you know, retinal structure, but they’re not quite the right shape, and they’re hard – theyre hard to manipulate or to deliver. What we’d really to do is get them to stand up and pay attention and do what we want them to do.” And they say, “No problem.” And the make us things like this.
And so, this is –
[return to the slide of the microscopic photo of custom photoreceptor scaffolds]
– this is an electron microscopic view of one of the scaffolds that was especially customly – custom designed for us. And each one of these little holes you see there is a – is a receptacle for a photoreceptor cell.
[slide with a photo of the custom scaffolds at a lower magnification with smaller photos of the three inventors: Jack Ma, Sarah Gong and Yei Hwan Jung]
And this was not easy to do; It’s done by Yei Hwan Jung, and with Sarah Gon and Jack Ma in her laboratory. And this is kind of what it looks like on a little bit lower magnification. It’s flexible. We can cut out – this is probably enough to treat hundreds of patients, and its actually – it is pennies to make with the manufacturing process that they developed.
[slide with a photo of photoreceptors in the scaffolds with the scaffolds outlined in the image]
And so, here’s a – you can’t see the actual scaffold. We’ve kind of removed that from -from image manipulation. But each one of these red lines is a photoreceptor that has a particular process that’s oriented correctly in it. So, we’re able to seed them into each one of those little wells, and we’re able to coerce them to know which way is up and which way is down.
So, this would not be a first line treatment, because using a scaffold is a whole another series of F.D.A. headaches.
[David Gamm]
But if we can get this working well, then we’re positioned to move quickly from our baseline treatments to something that perhaps more sophisticated and more likely to restore meaningful vision.
So, I’m kind of wrapping up. You guys are probably happy about that, but a couple other things that I want to – to mention are, as I said earlier, you know, whose stem cell do we use? So, we have i.P.S. cells, we have E.S. cells. E.S. cells obviously are – are not going to be from a particular patient, but i.P.S. cells can be. So, if we have a population, one thought is, “Well, we can make i.P.S. cells from every individual, so why don’t we just do that?” So, if a person needs a cell replacement –
[slide with a graphic asking the question Whose stem cells do we use? and if they should be personalized for every individual]
– we just personalize it for that particular person. The problem with that is sometimes you need them right away. Let’s say you had a heart attack, and you need cardiomyocytes. You don’t have two years to grow them in the lab, and it’s millions of dollars. So, if you’re not really, really, really wealthy, and you have a lot of time to wait, then it’s not really going to be very practical to do, especially not when you look at healthcare worldwide.
[slide graphic animates on the question of One size fits all?]
The other option is to say, “Well, one size fits all. Let’s have one cell that – that we can give it to anybody that has a disease.” Therein lies the problem with immune rejection. Because now you have a cell that’s not necessarily immune matched to the recipient that’s going to get it. And so, that means you probably are going to need some immune suppressive drugs, which in elderly population could be a lot of problems. Not that, that might not be the best way to do it, and certainly would be the simplest and most economical, but we’re kind of look – looking at an in between strategy –
[slide animates in the statement of Banks of partially matched cells]
– and that is to develop a series of cell banks, about 85 to a hundred different cell banks that are immune matched to large segments of the population. So, with about five specially chosen i.P.S. lines, you can match almost 20% of the U.S. population, like you would for a kidney transplant. So, not perfect. So, not every – it’s not exactly like that person, but it’s close, like you might, you know, look for a good match or a beneficial match for a solid organ. And if you move it up to 85 or a hundred cell lines, you can cover over 95% of the population.
[slide animates on the logos for Opsis therapeutics and Cellular Dynamics International]
And that’s the strategy that Opsis Therapeutics and Cellular Dynamics International here in Madison is adopting. So, they’re working with the Blood Center Wisconsin to produce these banks, so that they’ll kind be kind of an off the shelf. So, a person walks in, and you say, “You’re closest to line 42.” And so, you start there.
[David Gamm]
We don’t know, however, whether that’s necessary or sufficient. But for us, that’s what we’re – were gearing towards, because we think that it’s a nice bridge between dealing with the bio-biologic problems and dealing with the economic problems.
So, in terms of actual stem cell trials or what’s going into patients these days, the eye is, as I said, leading the way. Almost all the stem cell trials right now going on humans are in the eye, and they’re all with the retinal pigment epithelium. Why? Because, as I said way in the beginning, they’re easy to make and they grow in large swaths. They can be purified easily, and then they can be placed into the subretinal space. And theyre – they don’t have the same orientation problems that the photoreceptors do. They need to be oriented, but they tend to grow oriented. The down-downside of it is R.P.E. don’t detect light. So, when they – when they die, the photoreceptors then die afterwards. So, if you just replace the pistons and the – and the carburetor is missing, or the catalyst converter is missing, then you’re not really going to get the engine to start, right? So, you’re only replacing one of the two cell types.
[slide with logos of companies that have approved clinical trials involving R.P.E. derived from human E.S. and i.P.S. cells]
So, California Project to Cure Blindness has an – has an E.S. cell-based R.P.E. clinical trial that’s ongoing. Advanced Cell Technology, which is now Ocata, now Astellas, also has one. Riken in Japan is starting up one again with i.P.S cell derived R.P.E. And the National Eye Institute, Kapil Bharti, a friend of mine, is also doing one with i.P.S. derived R.P.E. So, a lot of energy is being put into putting the R.P.E. cells into patients to see if they will help individuals with R.P. and A.M.D.
[slide with a photo of photoreceptors asking the question of photoreceptor replacement]
But that leaves out what I think are the real critical players in this, and that’s the photoreceptor cells that were the – the subject of most of my talk.
[slide with the National Eye Institute Audacious Goals Initiative title and the stated goal of Restoring vision through regeneration of the retina with the Goals logo and the N.I.H logo]
The good news is that, you know, we’re making good progress enough that, not – not here – not only here at U.W., but around the world, but the National Eye Institute recently established their audacious goals for the next 10 years. And they feel strongly enough that this can- that this will actually occur, that they’ve kind of put their – their – their money down, and said, “This is actually what we’re – were looking for over the next 10 years.” And that is to be able to restore vision through regenerating cells of the retina, particularly photoreceptors and also Ganglion cells.
So, that’s very encouraging. And it’s an audacious goal, which means it’s not easy –
[David Gamm]
– but it also shouldn’t ridiculous. So, we got a long way to go, but it’s not so far-fetched that they would look silly at the end of 10 years, because there’s no possible way that they can attain that goal. And so, we at the University of Wisconsin, and there’s – theres the website there if you want to read more about the audacious goals. I recommend folks do that.
And we at the U.W. are playing a prominent role. So, at the McPherson Eye Research Institute, we have a number of members.
[slide with the logos of all the members of the N.E.I. Audacious Goals Initiative]
We have two large grants that we’re working on with University of Rochester, Johns Hopkins, and Harvard, to band together large teams with specific expertise to try to overcome these hurdles, not just of cell production, because we’re getting – moving along quite well on that, but also cell installation, making those connections and restoring vision, and making it a – a meaningful restoration of vision.
[slide of Managing Expectations with silhouettes of a baby, a man and a man running]
We have to manage expectations along the way. Super computers weren’t made overnight. We had little clunky things that took up whole rooms.
[David Gamm]
And then over the course of – of – of decades, we ultimately have our little cellphones now that have – that can do more than those whole room computers could do at the beginning. And similar to this type – type of technology, we have to crawl before we walk before we run. And so, when I talk to patients, I’m always quick to say, “You know, I really – you can’t really expect to go from blindness to reading the New York Times. It’s going to be an iterative process where we learn along the way. And if we ask the right questions, and we’re careful about it, and we’re – and were safe about it, that’s number one, we’ll be able to make those steps and hopefully arrive at a product that’s really meaningful in the foreseeable future.
And – alright, this – this is the bane of my existence. But, stem cells, they cure everything, right? So, and you can get on and – don’t go on the website and just – and just Google stem cells. You’ll get all kinds of things, and they’ll claim everything under the sun. And the rule of thumb is if they ask you to open your pocketbook-
[slide with A final word of caution with a snake oil logo]
– it’s probably a sham. Alright? At the state that we’re at right now with this – with this technology, we have to pay you, we have to search out the patients to come in and participate, and we’re going to pay for your travel, and we’re going to thank you profusely, and you’re going to get a gift certificate to Panera.
[laughter]
[David Gamm]
If they’re asking you to drop $40,000, then they have a conflict of interest. They’re making money off of you, right? And if the scientists that are really in the thick of it are saying, “We’re working, but it’s not a miracle cure, and somebody turns around says, “No, no, it’s a miracle cure for 40 Grand.” Then thats right – thats all the bells and whistles and flags should go up. There are plenty of very, very wealthy people in this world that are blind or have low vision. If there was a way to pay to get your vision back right now, they would do it. So, don’t fall for those – those traps. And that’s the best way to – to sniff them out. If they ask you for money, don’t trust them.
So, just to finish up here –
[Summary Slide]
– before I thank all the people that actually do the work, the technology exists to build human retinas in a dish. That’s amazing. That gets me up every day. And like many areas of stem cell technology, UW-Madison is – is a leader in that field, and the McPherson Eye Research Institute is – is playing a huge role in that.
Installing these new cells that we can make and that we’re so proud of ourselves about still remains a -a large challenge, but the expertise and resources and all the accoutrements that are necessary to really address these big challenges are here on campus or with our collaborators. And more importantly, the atmosphere is such that we like to work together. We like to take what I know best and meld it with what somebody else knows best and come up with better ideas and build a better mouse trap, and it makes it fun.
[Credit slide dissolving in photos of Gamm Lab scientists]
So, to finish up, here are credits. These are the actual people that I want to make sure that get highlighted. My laboratory as changed a lot over the years, and the people have been wonderful. They work very, very hard, and they’re brilliant.
[slide with photos of Beth Capowski, PhD and Joe Phillips, PhD]
Beth Capowski and Joe Philips deserve special recognition. Beth did a lot of the work on the three-dimensional structures that you saw, and Joe runs our transplant program. Without these two individuals, there’s no way I would be anywhere close to where we are right now.
[slide with the words Gamm Lab in a green circle that animates smaller and is intertwined with other smaller multi-colored circles with lines connecting them under the logo of the McPherson Eye Research Institute]
And, of course, my laboratory is just one of hundred plus that are part of the McPherson Eye Research Institute. I have them all interconnected here, because we talk with one another, we exchange ideas, we exchange resources and expertise. And that allows us all to move ahead faster and -and safer, and that’s the important thing.
[side with interconnected circles dissolves to the motto of the McPherson Eye Institute]
And so, kind of, our motto is to pioneer science, medicine, and art of saving sight. We’re very serious about our support of art. Because ultimately, what are we trying to save? We’re not trying to save the ability to see a white dot on a black background. We want to save or restore the ability of people to appreciate the visual world around them.
[slide of motto changes to photos of all the scientist collaborators]
And I have collaborations across campus. These are just some of them. Jamie Thomson is there, Bill Murphy, Sarah Gong. I can’t name them all, but just tremendously talented people that are willing and enthusiastic to work with us.
[slide with logos of all the organizations that support their work]
And, of course, we have a lot of sources of – of support that I’m very grateful for, I wanna, particularly, mention Dr. Monroe and Sandra Trout, and David and Nancy Walsh who have been very instrumental in getting a lot of this work going, as well as our other sources of funding.
[Thank you slide with shot of State Capitol as seen from the Lake Monona]
And with that, I want to thank you for listening to that. And I’ll take any comments and questions you folks have.
[applause]
Search University Place Episodes
Related Stories from PBS Wisconsin's Blog

Donate to sign up. Activate and sign in to Passport. It's that easy to help PBS Wisconsin serve your community through media that educates, inspires, and entertains.
Make your membership gift today
Only for new users: Activate Passport using your code or email address
Already a member?
Look up my account
Need some help? Go to FAQ or visit PBS Passport Help
Need help accessing PBS Wisconsin anywhere?
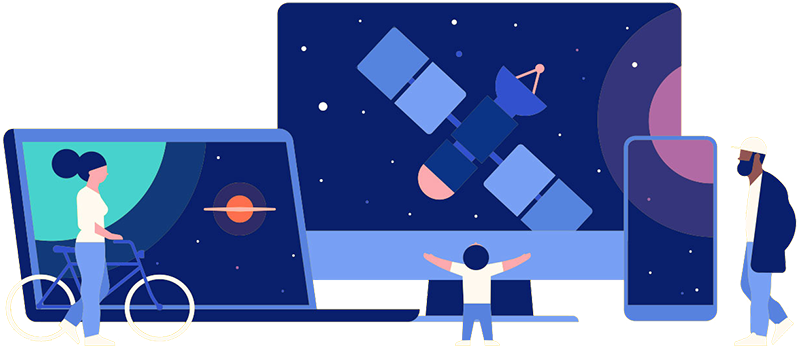
Online Access | Platform & Device Access | Cable or Satellite Access | Over-The-Air Access
Visit Access Guide
Need help accessing PBS Wisconsin anywhere?
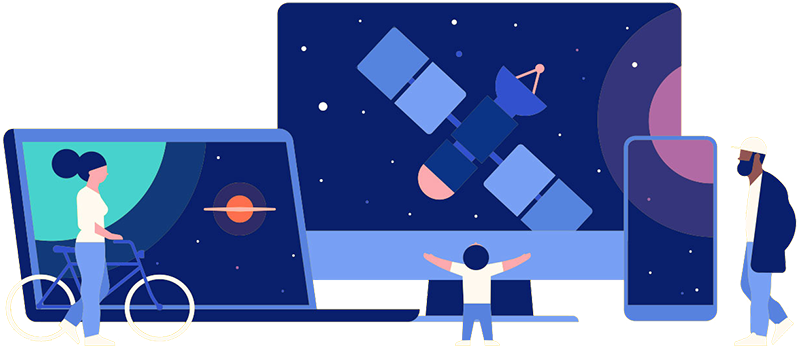
Visit Our
Live TV Access Guide
Online AccessPlatform & Device Access
Cable or Satellite Access
Over-The-Air Access
Visit Access Guide
Follow Us