– Hello, and welcome to Wednesday Nite @ the Lab. I am very excited to be here. Again, my name is Ellie Feitlinger. I work for Wisconsin IceCube Particle Astrophysics Center. And outreach and events coordinator. I’m super excited to be here to learn a little bit about IceCube, the biggest and strangest detector in the world. And I’m really excited ’cause we get to hear from Justin Vandenbroucke, and actually, a little bit about Justin. He is an assistant professor at UW-Madison Wisconsin IceCube Particle Astrophysics Center. He’s actually based primarily in the physics department with a joint appointment at astronomy department. A little bit about him though is really cool. He received his PhD actually at UC-Berkeley and eventually moved here to Madison in 2013. In addition to his work at IceCube Neutrino Observatory, Justin leads construction of a camera for detecting the highest energy photons in the universe and is also the leader of the Distributed Electronic Cosmic Ray Observatory, DECO, Citizen Science Project, which enables users to detect cosmic rays with their phones. So, let’s join and welcome Justin Vandenbroucke as he talks about Ice Cube and all of our discoveries.
(clapping)
– Okay, good evening, thanks, Ellie. Thanks to all of you for coming. I’m also really excited to be here. I’ve been waiting most of my career to be able to announce some news like this. It’s really exciting time for our field. So I’m going to try a little bit of an experiment tonight in science and in physics and astronomy. We don’t often tell stories in chronological order, but I’m going to attempt to do that here. You can tell me how well that experiment works or doesn’t work, so I’m going to borrow some techniques from other storytelling disciplines like theater. So we need to start our storytelling by introducing who the main characters of the story are and then setting the stage and the when and where it happened in addition to who. So starting with who, we have four main characters of the story, starting with this giant galaxy across the universe. That’s part of the title, this is called a blazar. It’s a giant black hole, I’ll tell you more about that later. Another main character, you’ve heard about from Ellie briefly, is the Ice Cube Neutrino Observatory at the South Pole, another main character is this NASA satellite in orbit around the Earth. That’s called the NASA Fermi Gamma-Ray Space Telescope.
And the final and the fourth witting character in the story is called the MAGIC Gamma Ray Telescope. These are both gamma ray telescopes Fermi detects high-energy gamma rays. Magic detects very high energy gamma rays, even higher energy from the Earth. So now you know who is involved in the main story. The next question is when and where it occured. So, the answer to that is like when and where many of the best stories occur, and that is a long time ago in a galaxy far, far away. So that’s, the stage is set now, we can dive into the story. Okay, but to characterize, this is still astronomy and physics so we still need to be quantitative. So what do we mean by long, long ago and far, far away? So let’s give a brief review of distances and times in astronomy. So, I think you’re all on the same page. To start off with, a year is a unit of time. We’re all familiar with that. But then, how do astronomers measure distance? Once you have the time, being one year, the way we measure distance is actually also based on that unit of time, so we talk about light years. That might be confusing because a year is a unit of time. A light year is a unit of distance. So, it’s a very convenient distance. It’s just equal to the distance that a light travels in one year. Light year is a unit of distance equal to the distance that light travels in a time of one year. We’re going to talk about neutrinos today also. Neutrinos because they have very, very small mass. They also travel at the speed of light. They travel the same distance in the same time as light.
So that’s our basic building block of both time and distance in astronomy. We can also make that much longer and much shorter by just multiplying by large and small numbers. So a year is kind of a medium distance of time. A light year is a pretty long distance of space in astronomy because we have such large distances. We use this large building block to measure distances. We need even larger distances than light years, so we have a billion light years. That’s obviously a billion times a single light year. So a billion years is a very long time. A billion light years is a very long distance. So we kind of have this obvious scaling relations. We can also go in the other direction, and that’s convenient because we can just use different units of time. So a minute is a short time. It should be singular, one minute, as a short time. And we can also just again scale by the speed of light. So a light minute, now depending on your perspective, that’s either a long distance or a short distance. By astronomy standards, that’s a short distance. By most of our standards, that’s a pretty long distance. So a light minute, is an eighth of the distance all the way to the sun, so it’s still a very long distance. Light travels one light minute distance in one minute of time. Okay, so now we have kind of a time scales and distance scales to go forward. Okay, so starting early on, I’ll skip over to Big Bang, but we’re still going to start pretty far back. Soon after the Big Bang, galaxies started to form. So a lot of you have seen this picture. This is called the Hubble ultra-deep field image. This is a large number of galaxies in a very tiny patch of sky, very, very far away.
So this is now five to 10 billion light years away. And the universe had already had enough time in the first few billion years of its existence to form these massive galaxies. Not only that, but today, we know that most of these galaxies have large black holes at the center of them, so an amazing fact. When I was getting excited about astronomy first, 20 years ago in high school, I was reading Stephen Hawking’s books, and he was talking about how black holes were amazing theoretical constructs, but who knew if they were a reality or if they would ever be detected. Now we know that basically, every galaxy that we’ve looked at has a giant black hole at the center, that includes our own Milky Way. And most of these are very massive. Millions or even billions of times the mass of the sun crammed into a single black hole at the center of every galaxy. So we call that a galactic nucleus. Kind of analogous to the nucleus of a cell. It’s a massive thing at the center of the cell, and even though it’s smaller, it has very important mechanisms to interact with the entire galaxy, even though it’s relatively smaller mass and volume at the center. Okay, so another fascinating thing about black holes is that even though they’re so massive and so dense that light cannot escape from them, there’s this amazing paradox there. Despite that, they’re actually the brightest objects in the universe, how’s that possible? It’s kind of like if you pull the drain on the bathtub and the water spirals down into it, there’s matters spiraling down into these black holes, and it’s spiraling so strongly that it’s glowing up and it’s heating up, and it’s being accelerated.
And so it’s generating huge amounts of matter and radiation but actually escaping and not falling down into the black hole, but they’re actually being accelerated. and that’s fundamentally because of the gravitational force pulling all that matter in a spiral down into the gravitational well. And so a fraction of it, after being accelerated doesn’t fall all the way in, but it shoots back out again. And often, it shoots out in these jets. So you can see that this is the artist picture, that jet coming up out of the black hole to the upper right, is basically a particle accelerator that’s being accelerated by the black hole. So kind of like the particle accelerators that humans built here on Earth, but it’s even more energetic reaching even higher energies of particle acceleration. So black holes are actually some of the brightest objects in the universe. Okay, so we’ve got some of the building blocks of our terminology now. You know what a galaxy is, it’s like the Milky Way. But other galaxies are much farther away. You know, what the nucleus of a galaxy is. That’s that black hole, the center of every galaxy as far as we know. And then there’s another third term that’s important here, and that’s an active nucleus of a galaxy. Some of these black holes are just sitting there at the center of the galaxy.
Some of them have a lot of matter falling down into them. And spewing out these accelerated beams of matter and radiation, those are called active as opposed to quiet or quiescent black holes. So you put that altogether and you get some jargon, active galactic nucleus, but it’s just got three simple pieces. So here’s a side view of that, now a cartoon on the left. You can see the black hole at the center. You can see the two jets spewing out perpendicular to the matter that’s spiraling down into the black hole. If you look from along the side, along the view of one of those jets from the right, you have a top view of the same situation. And at that point, this jet is pointed right at you. And so that subcategory of these active galactic nuclei is what we call a blazar. It’s basically like the barrel of a gun just pointing straight at you, having a particle accelerator being pointed straight at you. And so a subcategory of these galaxies with these giant black holes at the center have a particle accelerator pointed straight at Earth, and that’s what we call blazars. Okay, so now we’ve got kind of the basic background of what happened here, and now we know the time scales and the distance scales of all, so now we can start our story. So the story started long, long ago in a galaxy far, far away, and now we can say exactly how long ago and how far away. And the answer is it happened four billion years ago, and the information about it just reached us.
It happened four billion years ago, so the place that it occurred, the distance it occurred was four billion light years distant from the Earth. So that’s when the story begins. So a particular one of these active galactic nuclei, that was also a blazar, emitted a neutrino. So we’ll come to what a neutrino is, but just keep in mind that the distance and the time scale to begin with. Okay, I’d like to go through all of the last four billion years now, but I know you guys have other things to do, so I’ll try to compress it into an hour. So we’re going to pay attention to the next minute, cause we’re going to fast forward a lot very quickly. So what happened over the last four billion years? Okay, four billion years ago, starting point of the neutrino was emitted. It was born at that massive black hole. A little bit later, 3.8 billion years ago, life began on Earth, the Earth had been evolving as part of the solar system until that cooling down from its original formation. Fast forward more, this is an important part of our story later on. Only a billion years ago, there were two black holes– These are much smaller than the ones at the center of the galaxies– but two black holes basically collided and merged with one another. Okay, continuing with the story here on Earth, half a billion years ago, fish evolved.
0.4 billion years ago, land plants evolved for the first time. 0.2 billion years ago, dinosaurs and mammals both evolved, roughly around the same time. Dinosaurs and mammals here on Earth. Another important astrophysical event, 0.1 billion years ago, two neutron stars merged. So these are like black holes in the sense that they’re very massive and very dense, not quite as dense as black holes. So light can escape from them, but they’re basically like the giant nucleus of an atom, similar density but on the scale of an entire star. Okay, now things are a little more interesting for humans. 0.002 billion years ago, Homo sapiens evolved, and that included astronomers. You have to have a lot of digits here. So this is, if you count the zeroes, this is 0.2 million years ago, or only 200 thousand years ago, tiny fraction of the time that this neutrino had been traveling across the universe. I say astronomers were included in the Homo sapiens because a lot of those Homo sapiens looked up at the sky and so they were doing astronomy, especially the ones who got more systematic than just looking at it, but they tried to make systematic observations and write down when things appeared and when they changed and so on. Okay, another important milestone for the astronomy side is that a star in the large Magellanic cloud, a nearby galaxy, exploded at the end of its life. This also happened at the same time that the first humans and the first astronomers appeared. Okay, more recently, about 2000 years ago, a particle physicist evolved, and notice that they evolved more recently than the astronomers. (laughing) You can take from that what you want to.
Okay, so I’m counting as particle physicist, the Greeks basically, there’s one, especially Democrates, who had a theory of atoms, and he believed that matter was made fundamentally of particles. If you don’t count Democrates as a particle physicist ’cause he didn’t have his own accelerator, you can wait until about 40 years ago when modern particle physicists evolved. And so they had giant particle accelerators. And that’s when basically our modern understanding of particle physics arrived, and that’s called the standard model of particle physics. Okay, so what is that standard model of particle physics? Here it is, I was pretty bad at chemistry. The periodic table was way too big for me, and I had a terrible memory, so I really liked physics, with its very small version of the periodic table. It’s really simple, they’re only a few building blocks. And in fact, you can actually ignore most of this. So what is matter made of? It’s made of atoms, the atoms are made of nuclei consisting of protons and neutrons and also, the atoms have electrons surrounding them.
So we have the quarks that make up the neutrons and protons. Those are the up and the down quarks. And then we have the electron. So if you only care about normal matter, about the pieces of normal matter, you can basically only pay attention to these three. Here, we care about more than just the building blocks of normal matter. So it gets a little bit more complicated. In addition to these three pieces the matter is made of, there’s also a neutrino, which is associated with those. So it’s also a kind of piece of matter, but it’s not what matter is made of. It comes out from interactions between matter. Nuclear interactions between matter. And it’s a very important part of how the sun shines. So it is an important part of the behavior of matter, just not what matter is really made of. And then a really kind of strange and a thing that’s not totally understood today, is that we basically have three copies of that same strip of particles which is repeated three different times. So you have that up, down electron and electron neutrino on the left column, and then you repeat that two more times and that includes this muon and the tau. And so the muon is basically like a very heavy version of the electron, very similar physics to the electron but a much heavier version. That muon is also an important part of the story here.
Okay, so I focused on the particles that are in black for this talk. We have on the left, the parts that make up normal matter. In the middle is the muon and the muon neutrino. These are basically heavier versions of the electron and the electron neutrino. And so they organize into these different columns. And because neutrinos have very little mass, they basically travel at the speed of light, very, very slightly below the speed of light. Okay, that brings us to another amazing fact of particle physics, which is that at the very highest energy, very highest energies of light, light is actually absorbed by other light. Something we never experienced in everyday life. But imagine you’re in a dark room, and you turn on a beam of a flashlight, you turn on another one, and you make their beams cross and one beam is blocked by the other beam. Something you would never have any intuition with in everyday life. But we’ve measured it in particle physics, and we know that that occurs. We’ve also calculated it, and so it all agrees with our understanding of modern particle physics. So, the very highest energy type of light is absorbed by crossing paths with lower energy types of light. And that’s an important problem because for the frontiers of physics and astronomy, we want to understand the highest energies and the longest distances, and we can’t do that with light because of this, so if you go to the highest energies and longest distances, light simply cannot travel across the universe. It’s blocked by other light, by low energy starlight.
So we need something else other than light. And one thing we can use for that purpose is neutrinos. They’re kind of like super X-Rays. X-Rays, you’re familiar with from medical imaging and other applications, they travel straight through a lot of matter, so they let you image the interior of objects. Similarly the neutrinos travel straight through the universe including through matter and light. So they’re not blocked by other light or by other matter. Okay, so where are neutrinos produced? They’re not part of, they’re not a building block really of normal matter. Even though they’re made of matter, they’re produced by interactions, by nuclear and particle physics interactions around the Earth and around the universe. So, this is a graph of, you can think of it as how likely a neutrino is to collide with an atom on the vertical axis as a function of how energetic the neutrino is on the horizontal axis. So they were produced abundantly in the Big Bang, starting all the way on the left. They’re produced by radioactive reactions, both man-made reactors, also naturally occurring ambient radioactivity, terrestrial radioactivity. They’re produced in the sun as part of the fundamental mechanism of how the sun shines. They’re produced by exploding stars, supernova. And they’re also produced when energetic particles hit the Earth’s atmosphere and trigger particle physics interactions naturally high in the Earth’s atmosphere.
We get a bright glow of neutrinos from that. We can also produce them artificially not only from reactors but also using particle accelerators on Earth. And what we’re interested in in the field of neutrino astronomy is a few of these, especially the very highest energies, but starting off at medium energies, the sun and the supernova, they glow in neutrinos. But what I’m going to focus on for IceCube is the very highest energy range, where there’s galactic and extra galactic neutrinos that we think are produced by particle physics interactions throughout the universe. Okay, to give you a benchmark scale for this interaction probability on the vertical axis, neutrinos at medium energies can travel huge amounts, huge distances through dense matter. So even if you have lead, one of the best materials for absorbing X-Rays and other particles. Even if you have a light-year of lead, a neutrino can travel all the way through it at these intermediate energies. If you go to even higher energies, they’ll travel shorter distances, but that’s the scale at medium energies. Okay, how do we detect the neutrinos? We use Cherenkov radiation.
So, a good analogy for what’s Cherenkov radiation, if you have a boat or a duck traveling through the water, and you don’t see that boat or the duck but you see the wake of it, you can basically triangulate and say there must be a boat or a duck here because there’re some waves that are propagating with this triangular pattern from a point that’s right here. The same thing happens with charged particles that are traveling through matter. So they make effectively a wake of electricity in their path and so if you detect, and that wake of electricity produces visible light, blue light and ultraviolet light. And so in the case of energetic particles traveling through matter on the right, that’s actually a picture of a nuclear reactor which is emitting a lot of these particles, and they’re glowing in this blue light, which is the Cherenkov radiation, analogous to the wake of the duck. And so you can figure out there must be a, energetic particle or a neutrino that produced that. Okay, so this is now the foundation of what we call multi-messenger astronomy. So ever since those first Homo sapiens, those first astronomers started looking up at the sky, they were doing astronomy with different types of light. They expanded to other types of light, including energetic light, like X-Rays, low-energy light like radio waves, but those are all different types of light Now with particle physics, we can use other types of particles to do astronomy, rather than only light, and so we can use cosmic rays, these are energetic nuclei that are accelerated in these large, naturally occurring accelerators, like the black hole that I showed earlier.
We can also use neutrinos as messengers to do astronomy. And finally, we can use gravitational waves. That’s another exciting, new part of this field of multi-messenger astronomy. There’s a lot of different science we can do with this new type of multi-messenger astronomy. One of the most important topics that we can study is the origin of cosmic rays and what their sources are. So we know that they’re these very energetic particles we call cosmic rays, hitting the atmosphere and hitting the Earth all the time. We don’t know exactly what’s producing them or how it’s producing them, but we think that those sources– Here’s a cartoon on the upper left again of this galaxy with the jet of matter and radiation coming out of it. We think that that’s producing not only cosmic rays but also types of light and types of neutrinos. And so by studying those, we can understand the sources and the accelerators out in the universe. And it has, in the case of neutrinos, has this advantage of being a kind of super X-Ray that can go straight through any intervening matter or any intervening light, even though the high energy light would be blocked by what’s in between us and the particle accelerator. Okay, so that’s the idea of multi-messenger astronomy. So how does neutrino astronomy, how does neutrino astronomy work? Here’s a cartoon of what’s going on in the atmosphere when one of these particles, these cosmic rays hit the Earth. So you can see for example a proton coming from outer space as a cosmic ray colliding with the atmosphere, and it undergoes a particle physics interaction to make a bunch of particles. Some of them are absorbed in the Earth, but some of them travel all the way down, absorbed in the atmosphere. Some of them travel all the way down to the bottom of the atmosphere and reach the ground and even keep going into the ground. And those are neutrinos and muons.
So those are some of the particles we’re most interested in. Now we can detect both of those with a detector like IceCube. We can detect both muons and neutrinos. One challenge is that we have a much higher rate of these muons compared to the neutrinos that we’re interested in, so we detect about 70 billion muons per year. We have to detect these atmospheric neutrinos, the neutrinos that are produced in the atmosphere as a very tiny fraction of those, about one in a thousand. And then out of those, we’re looking for the astrophysical neutrinos that started above the Earth’s atmosphere, out in these cosmic sources. So that’s only one in 10 to the eight out of those atmospheric muons. And so we have to find a needle inside a haystack, and so it’s a big experimental challenge. And of course, that’s on top of the fact that these neutrinos are very unlikely to interact with matter to detect them. Okay, coming back to our timeline and our story. So, 31 years ago, after kind of this original idea of developing the field of neutrino astronomy, people-built detectors that could use Cherenkov light to detect the neutrinos. And they built one in Japan called the Super-K detector. And if you remember that star that exploded, around the time that humans were first evolving in the large Magellanic cloud, the humans had evolved, the particle physicists had evolved. They had built this Cherenkov detector.
And then that burst of neutrinos from that exploded star arrived at that detector, so that happened 31 years ago. So that was one milestone in neutrino astronomy, that we could detect this first source of extra terrestrial and extra galactic neutrinos. You could argue that this is not neutrino astronomy because those detectors couldn’t figure out where the neutrinos were coming from exactly. They knew they were from this supernova because they saw the supernova in bright light at the same time but they were using timing rather than direction. Okay, continuing forward with neutrino astronomy, there was another milestone where another astronomical object was detected 20 years ago and this is the image of that. Any guesses of what this astronomical object is?
– [Audience Member] Sun?
– The sun, yeah. I mentioned there were two medium energy sources of neutrinos, the supernova and the sun. So this is an image of the sun and neutrinos. The sun is actually much smaller than you can see here, not because the neutrinos are glowing in a halo much bigger than the sun, but just because of the angular resolution of the detector. So they can’t actually image with the same resolution as the emission region of the sun. But at least you can say if they’re coming from in that direction in the sky, and that is where the sun is. Okay, moving along in the timeline. 27 years ago, it was proposed to do this in ice rather than in water, and the detector that was led by the University of Wisconsin along with a couple of other universities, they installed some sensors into Greenland ice, and they detected Cherenkov light from muons from the atmosphere. So they proved that you can do this technique in ice, not only in water. That was an important milestone led right here at UW-Madison. 17 years ago, another mile, another important milestone. They realized that the ice was even better at the South Pole than in Antarctica, it was even clearer, and there was more of it, and so they installed an array of detectors. You can see it’s a bigger group of collaborating institutions and funding agencies at this point, but it was especially enabled by WARF here at UW-Madison.
So they built a detector called Amanda, and that Amanda detector at the South Pole detected neutrinos for the first time using this Cherenkov detector in the ice. So it’s just a flash of blue light due to a neutrino colliding with the ice. And those neutrinos now were coming from the atmosphere. So they’re not just those background muons that were easy to detect, now we’re detecting neutrinos from the atmosphere. But they’re still background in the sense that they’re not the neutrinos coming from outer space. They’re still from the atmosphere. That was 17 years ago. Okay, so that brings us to our second player in the story. We have the super massive black hole, the blazar. And this is now the IceCube Neutrino Observatory. So this is not to scale, but there’s a big detector in the middle of Antarctica, this is now the successor of the Amanda experiment, a bigger version. It’s sitting right at the South Pole, in the middle of Antarctica, and it’s using a large volume of that ice as a detector for this Cherenkov light, why ice? Because you can straight through it. It’s one of the clearest naturally occurring large volumes of material on Earth, so that you can monitor huge volume of ice for this very rarely interacting neutrinos. Okay, so this is the ice, diagram of the IceCube detector. It’s basically an array of individual light sensors. They’re about 5000 individual light sensors.
And continuing with our timeline, this detector was completed about 10 years ago. A huge effort by a lot of people around the world, a really successful construction project that culminated in 2010. So it’s a simple, pretty simple concept but very difficult engineering to achieve, installing 5000 different light sensors, spanning a cubic kilometer of ice, monitoring a billion tons of ice for this neutrino interactions. Here’s how the detector was constructed. It’s again a simple concept, but an enormous engineering challenge, and this was a success thanks to the physical sciences lab here, in Stoughton, just outside of Madison. So the idea is that you heat up water to very high temperature and very high pressure, and you squirt it on the ice. And then you make a hole, you lower it farther, you keep squirting, and you just do that for a couple of days, and you drill down a mile and a half by doing that. And then you’ll just do that 86 different times over seven years. (laughing) Really, again, really simple concept, a very huge engineering challenge. So there’re about 50 drillers on this team working around the clock in harsh conditions at the South Pole to pull this off.
Here’s just a movie of what that process looks like. A cartoon, so squirting that hot water, First you start with kind of a hot butter knife approach because there’s a layer of snow on top. If you squirt hot water that’ll just seep out into the edges. Then you pull that hot butter knife approach out again and go with the hot water hose approach. And now this is the fully-densified ice, that’s been compactified after it fell as snow at the top, and it’s just solid ice. Squirting that hot water and just continue squirting it all the way down a mile and a half deep in the ice. So those holes are a mile and a half deep and about 67 centimeters across. And it’s hard to have a feeling for what those numbers are like until you’re standing over one of the holes and looking down, and they’re just barely wider than my shoulder. So I had the chance to work there for three seasons during construction, a lot of fun but a little bit scary to stare down one of those holes. (laughing) Here’s what it looks like from the top. So imagine a mile and a half all the way down to the bottom of the ice there. Here’s what it looks like from the surface. There’s the new South Pole station. This is the Amundsen-Scott National Science Foundation South Pole Station, so that’s all here. It houses scientists and support staff. There’s a runway where airplanes can land and take off. And then across that is where IceCube is. So it’s deep under the ice here, a mile and a half down, but there’s a computing building at the top where all of the data come.
Okay, so this is a large collaboration. This is big science, and it takes a huge team of great people to pull off all of this engineering and science. As of today, consists of 12 countries on four continents. It’s about 49 different universities and labs around the world and we have about 300 scientists who are collaborating together. Why is IceCube so deep at the South Pole? It’s buried a mile down. First of all, you need a large volume, but even that large volume actually displaced down in the ice, we don’t instrument the top of the ice and that’s for a couple of reasons. The ice at the lower depths is actually bubbly. It’s more like the ice that you would get in your freezer. It’s kind of milky and opaque because it’s got little bubbles. But if you go deeper, if you go at least a kilometer down, it’s crystal clear, and you can see for hundreds of feet through the ice. And that’s what you need to detect this Cherenkov light. We’re also using the upper layers of the ice as a shield for this background muons. Remember we’re more interested in the neutrinos than the muons. And so those muons are absorbed by the upper part of the ice. Okay, there are two different types of signals that we detect in IceCube, one is what we call a track and one is what we call a cascade. And you can see they look different, they have different shapes. I won’t get into the technical aspects of it, but they have different advantages and disadvantages in terms of the science that we can do with them. But they’re both signals that are produced by neutrino interactions in the ice.
Okay, so continuing with our timeline, we were constructing IceCube 10 years ago. We completed it about seven years ago. And very soon after we completed construction, we made the first discoveries. We discovered the first astrophysical neutrinos. Remember these milestones, atmospheric muons, atmospheric neutrinos, now finally, astrophysical neutrinos. That was on the cover of these two scientific journals that are important in our field. You can see the pictures of these, of these depositions of energy from these neutrinos interacting in the detector. Remember this is a huge volume, right? It’s a kilometer across and these neutrinos are so energetic even though they’re a subatomic, fundamental particle much lower mass than an electron, they still have so much energy that they light up this entire kilometer across of light instrumentation. If you want to know what a cubic kilometer across neutrino interaction looks like for human scale, you might recognize this landmark that its overlaid on, on top of, this is the Memorial Terrace, and this is Lake Mendota, and this is a large fraction of UW Madison campus and Madison. So you can imagine the single subatomic particle lighting up the entire sky over Madison. It’s got energy that– Each one of these neutrinos has energy that’s about a thousand times more energetic than the largest energies we reach on Earth with human accelerators. Okay, where are they coming from? That’s the most important first question. We discovered that there is this glow of neutrinos from the universe. The next thing we want to do is actually do astronomy with them, answer the question what’s producing them.
So we do that the way astronomers answer that question, and you just make a map of where everything’s coming from. You image the sky in neutrinos rather than in light. And this is what we get. This is a simple projection which is just taking the Earth and projecting it out into the sky. And so the North Pole would be up on the top, the South Pole on the bottom. So the galaxy goes across that view. It’s marked in that as that solid black line, and you can see the galactic plane there. The first conclusion you can draw really simply from this picture is that they’re not coming from the galaxy. They’re not all following that line. So they must be coming from beyond the galaxy because they’re distributed evenly across the entire sky. So that’s a really important first conclusion about what’s producing them. But for the most part, we have not been able to figure out what’s producing them, that’s remained a mystery. Beyond this first step of saying that they’re evenly distributed across the sky, therefore, they’re beyond the galaxy.
We also did another technique. So this first technique was using what we call those cascades, that one spherical deposition of light that I showed you a few pictures of. We also use this other technique, which is when a neutrino converts into a muon inside the detector. And that produces this long, straight line of light, and we can do that, we can image neutrinos coming all the way through the Earth. Remember, they can travel through large distances of matter. The neutrinos travel through the Earth, then they interact in the ice to produce a muon, and then we can measure that muon in a long, straight track and calculate the direction of the original neutrino. That’s a powerful technique because we block out all the muons because the original muons from the atmosphere cannot travel all the way through the Earth. Only a neutrino could travel all the way through the Earth. So in this cartoon, we’re looking through the Earth in order to shield ourselves from the muons produced in the atmosphere in order to only detect neutrinos that can travel all the way through the Earth. So we did that approach, and we also discovered the signal again basically with the complementary method. Okay, continuing with our timeline and the story of multi-messenger astrophysics, if you remember this in that first fast forward, there was a billion years ago, two black holes that collided and merged, and when they did that, they launched ripples in basically in the fabric of space and time. And those are known as gravitational waves. Those spent the last billion years propagating across the universe, and they finally reached the Earth three years ago.
And at that point, the astronomers and the particle physicists had evolved highly enough that they could build a really good detector, and they were ready and they detected that ripple of gravitational waves. And so that was a huge discovery three years ago, and it got the Nobel prize last year. That’s an important part of the story of multi-messenger astronomy. Okay now, coming up to two years, we’re getting closer to the present. Two years ago, IceCube had basically established this diffused glow of neutrinos from the cosmos, done a lot of different analyses to try to understand what’s producing them, and that’s using combining the neutrinos with different types of other signals including cosmic rays and different types of light. And basically, we’ve been slowly ruling out individual types of sources, and so it’s growing as a bigger and bigger mystery of what’s actually producing these neutrinos. And so we realized a couple of years ago, we can do this better if we partner with our collaborating astronomers at other telescopes, not only just looking at data we’ve collected but to do it in real time. So instead of taking years of work, to analyze the data to figure out when and where the neutrinos came from, can we do that all within a few seconds rather than a few years. It was a big effort by a lot of people in IceCube to get this infrastructure up and running. We’re now, when a neutrino hits the ice, we can calculate the direction that it came from within a few seconds, and not only calculate it but release it to the world in real time and tell them when and where to look on the sky in case there’s something still interesting happening in that direction.
So we implemented that system two years ago, and since then, there’ve been 14 interesting neutrinos. Each one of them, within a minute of the time the neutrino collides with the Earth, IceCube automatically calculates where it came from and publishes a website where astronomers can basically say, “Let’s point our telescope in that direction.” It’s totally open, so any professional or backyard astronomer can point their telescope in that direction. Okay, we’re still in the meantime trying to figure out what’s producing these neutrinos. So a year ago, we published our most stringent search for that. And we basically do that by looking for clustering in individual directions on the sky. And so this color scale indicates clustering, if there’s any clustering of particular direct, in particular directions on the sky of neutrinos. And that would indicate an individual source of neutrinos. And even though your eye can pick out individual clusters here, none of them are significant statistically. It’s kind of like how you can see shapes in clouds, but they’re just random shapes. And so as of a year ago, we had still never identified a single source of this very high energy neutrinos. So we’re getting closer up to the present now, a year ago. Here’s a cool movie, showing those 14 different alerts that we’ve published to the web. So you can see over the last couple of years how they’ve occurred at different positions on the sky at different times, and so you can see, we’re detecting them from all over the sky and at a roughly random time along the horizontal axis.
Okay, that brings us to next milestone. If you remember in that fast forward timeline over the last four billion years, there was also a really astronomical event happened which is that after those two black holes merged, there were two neutron stars that merged. And that light traveled across the universe, and it finally just reached us 12 months ago. That was another really big milestone in multi-messenger astronomy. You’ve probably heard about that. That was exciting because not only gravitational waves were detected from that merging of two neutron stars but also flashes of light from across the entire electromagnetic spectrum, so for the first time, we’ve seen both gravitational waves and light from the same astrophysical event. So that brings us up to 12 months ago. Now we’re coming towards the culmination of this four-billion-year story. After that four-billion-year-old neutrino had traveled across those four-billion light-years of distance, it was really tired after that long travel. (laughing) It was finally ready to lay down to rest, and it collided with a molecule of ice inside the South Pole next to the– inside Antarctica in the middle of this– in the middle of Antarctica, near the South Pole, outside the IceCube detector.
It produced a very high-energy muon. That muon traveled through the detector, and it lit up the neutrino, it lit up the detector. So here you can see all the different light sensors in IceCube that were lit up by that detector. And you can see just by eye that if you draw a line through this energy pattern, the neutrino must have come from over here. The color here represents that it came first in this position, the red, and it came last in this position, the blue. And so we can do a more sophisticated than just drawing a line by eye, more sophisticated analysis. And we can figure out it came from this particular direction seven degrees below the horizon or six degrees below the horizon within one degree of uncertainty. We also measured its energy as a very high energy, again hundreds of times more energetic than the highest energy particles we’ve produced at accelerators on Earth. And so again this whole infrastructure was set up so that we were ready after this four-billion-year journey to detect this neutrino, so it collided with the ice that made the detector light up. The computers on top of the detector calculated what direction it came from. Those computers published a website to the public. It did all that within 43 seconds. And then some other humans read that website and said, “That’s really exciting. Let’s point our telescopes over there.” Some other telescopes didn’t even need a human. They just said, “That’s really interesting. We’re going to point ourselves over there without a human,” because there are actually some robotic telescopes today. So you might say that’s the next step in the evolution of astronomers.
Okay, so here’s what came out of that. So 23 different observatories around the world pointed in that direction. IceCube’s lucky because it can see the entire sky. A lot of traditional telescopes can only point at a particular direction in the sky. That’s why it’s really important to release this information within a minute. So that they can point in that direction while it’s still interesting. A lot of different telescopes around the Earth and also in orbit around the Earth, those ones on top of the Earth are in orbit, a lot of them pointed in that direction, and most of them detected something from that same direction. There’s something interesting going on in the direction of that neutrino. So, that brings us, we’ve gone through the blazar, we’ve gone through the IceCube detector. Those are the first two characters in the story. That brings us to the third character which is the Fermi Gamma-Ray Space Telescope operated by NASA, so that’s in orbit around the Earth. And it’s basically scanning the whole sky all the time. Scientists looking at that data pointed out that there was one of these blazars in the same direction as that neutrino. And so that’s how we identified that it’s likely that it was a blazar, this massive black hole, that’s what actually produced the neutrino coming from that direction, thanks to this NASA satellite, Fermi. That’s detecting high-energy gamma rays.
There’s another telescope on the ground, the MAGIC Gamma-Ray Space Telescope, which motivated by the IceCube Neutrino signal in the MAGIC high-energy gamma-ray, and the Fermi Gamma-Ray, high-energy gamma-ray signal, it searched for even higher energy gamma rays, very high energy gamma rays. I know we use technical language, but in this field. There are numbers associated with that, but MAGIC detected even higher energy gamma ways coming from the same direction. So the Fermi telescope identified that there was a known blazar in that direction. We already knew there was a blazar in that direction. But no one had ever detected a neutrino from it. And so that’s the first time that we’ve identified an individual source at these very high-energy neutrinos. Here’s just a map of what that looks like, a map of that region of the sky. You can see the Fermi data is in the color scale here. So purple and orange represents the brightest gamma ray emission from that, from different directions. You can see these contours indicate where we think the neutrino came from. So with high confidence, it was within this red circle. With medium confidence, it was within this gray circle. Our best estimate of where it occurred was at this green position, and this NASA satellite knew that there was this blazar at this circled position based on this bright distribution of individual gamma ray events from that direction.
Okay, so you might ask, there are a lot of these black holes on the sky, isn’t it possible that that neutrino just happened to come from the direction of one of those black holes, it’s just a chance coincidence. And that’s a really important question in astronomy because unfortunately, to astronomy, the world is basically two-dimensional, so everything is collapsed onto a projection of the sky, right. We don’t know for the most part, how far away something is that we detect. It could be that it came from something that’s just roughly the same direction on the sky, but it might be far behind or far in front of the actual source that we’re interested in, the black hole that we’re interested in. So we basically spent the next several months– A lot of astronomers in this field spent the next several months after September of last year calculating what’s the probability that this is just a random chance coincidence. And we estimated that it’s about one in a thousand. So it’s pretty unlikely that this is a false association that we’ve determined. But it’s pretty unlikely, and as scientists, we’d like to be more confident. So we’d like to be, like to beat that number down and say that it’s like one in a million to be, you know, you never prove it, you never have one in infinity but the higher you can make that number, the lower you can make the probability that you’re wrong about understanding what’s going on here.
So that’s what we want to do next, is basically increase our confidence that we understand what’s going on. We also know that there are– By doing a quantitative analysis, we know that there are at least 100 different sources producing the diffused glow of neutrinos that we’ve detected So this is just the first one that we’ve detected. So we want to understand this one better. We want to detect the other hundred or more that are out there. We want to understand what’s producing all of the neutrinos. We think that these black holes are actually only producing a small fraction of the total flux, the total emission of neutrinos. And so we want to understand what the other mysterious classes of sources are there out there.
Okay, but there were actually two– The story continues because there were actually two different papers that were published on the same day back in July. Some of you might have heard the– seen the newspaper articles and seen the headlines that were sparked by these two papers. The first one describes the story that I’ve told so far. And that was kind of old news for a lot of astronomers in the field because of these public webpages. So all these telescopes had been writing public webpages saying we identified something interesting from the direction of that neutrino. But there was another exciting part of the story which is that within IceCube, we had also gone back in our data, not only looking at that 2017 single neutrino that we detected but saying if there’s a neutrino coming from this giant black hole in this direction, what about the last decade of data that IceCube’s been collecting? Were there ever previous neutrinos from that same direction? And that’s a hard question to answer because of this very high rate of background nuance and background neutrinos from the atmosphere. But once you have a precise direction to look in, you can do a sophisticated statistical analysis, and we did that and we found there was an excess of neutrinos from that direction even before this 2017 arrival of this neutrino. So we detected a burst of neutrinos that happened. It lasted for several months, and it came from the same direction.
Here’s kind of a picture of what that burst looked like on the sky. It had only about 13 neutrinos associated with it. But that’s enough to be an excess above the background rate of other neutrinos from that position on the sky. So it lasted for about five or six months during 2014 and 2015, long before we detected this high-energy neutrino in 2017. So that boosted our confidence that we really understand what’s going on here, that there’s a black hole that’s producing all these neutrinos. But that’s just the first step. I should say that we don’t really understand because in this case, there were actually no gamma rays detected by the gamma ray detectors from the same direction at the same time. So there’s something very subtle and exciting going on here that we don’t understand yet. Okay, so to recap, here’s kind of the timeline of neutrino astronomy focusing on the story in Antarctica. This idea of doing astronomy using neutrinos, using Cherenkov light, using the huge naturally occurring ice at the South Pole, that idea first started in 1988. Then we had this predecessor detector, Amanda. That reached an important milestone of detecting neutrinos from the atmosphere for the first time, proving the concept of this technique. 2011, we finished building this large engineering and physics undertaking. An important milestone five years ago then the first of these neutrinos from beyond the atmosphere were detected. And finally just this year, we’ve announced the first identification of an individual source.
So I put a dot-dot-dot here because this is kind of still the beginning of neutrino astronomy. It’s the first time we’ve identified a single source of these very high energy neutrinos. It’s kind of like detecting a star for the first time in astronomy. So we think it’s the beginning of a new field of neutrino astronomy. So how do we go farther from here? We want to keep taking more data with IceCube of course, understanding the other hundred sources that we think are out there, understanding other types of sources beyond these giant black holes. But it’s pretty slow progress with these very small probability of neutrinos hitting an atom. And so we want to build an even bigger detector that’s called the next generation of IceCube, IceCube Gen 2. You can see in the middle here, that dense array, that hexagonal array of detectors in the middle viewed from the top is IceCube, and we want to surround it with additional light sensors surrounding it in order to have a bigger volume to collect these neutrinos. Okay, before I conclude, I just want to give one advertisement. Ellie mentioned this quickly. If you want to do particle physics, if you want to do astrophysics, you don’t need to build IceCube yourself or join us. You can actually use your own detector in your pocket. That’s called DECO, the Distributed Electronic Cosmic-Ray Observatory, and here are some pictures that we’ve collected with DECO.
The idea is that you run an app on your cellphone. When a particle travels through the camera sensor on your phone, it works very similar to IceCube on a much, much smaller scale. And it can detect individual particles. And so here are some examples, these pictures on the right are individual particles we have detected with DECO. And on the right is a picture of all the different places around kind of the region of Madison and the Midwest and the east where users are detecting these particles. You can download it at that website if you’re interested. Okay, so there’s a clich in multi-messenger astronomy which is that we’re opening new windows on the universe. But clichs of course are coming to become in place because they’re true, so I think in this new era of multi-messenger astronomy, we really are opening these new windows. It’s new ways of seeing the universe using all of our senses rather than only using sight. We’re also starting to use hearing and taste and all these different senses, feel maybe, beyond just looking, and of course, an analogy to our everyday experience, if you can do that in astronomy, then you can learn much more about the universe than just using one of our senses. So here’s a cool image of that, kind of taking the analogy literally. New windows on the universe.
These are stained glass windows on the universe. On the left, it’s a neutrino detector. In the middle, a gravitational wave detector, and on the right, a gamma-ray light detector. So to conclude, neutrino astronomy is providing a new view of the universe, it’s kind of this super X-ray vision of the universe. It’s giving us totally complementary information, new information beyond what we can do with light. And when we do this new type of astronomy, we found already that the universe is full of these other signals. It’s not just full of light, it’s also full of gravitational waves and neutrinos and particles. We’ve identified the first source. That’s an important milestone. But, we still have important questions about that source. We want to beat down that probability, that false probability to much smaller levels so that we can be really confident that we understand that this is a true detection, even though we think it’s unlikely to be an accidental detection. And we want to detect more of these sources and more of the classes of sources. And this new era of observing the universe with all of our senses is just beginning, thanks.
(applause)
Search University Place Episodes
Related Stories from PBS Wisconsin's Blog

Donate to sign up. Activate and sign in to Passport. It's that easy to help PBS Wisconsin serve your community through media that educates, inspires, and entertains.
Make your membership gift today
Only for new users: Activate Passport using your code or email address
Already a member?
Look up my account
Need some help? Go to FAQ or visit PBS Passport Help
Need help accessing PBS Wisconsin anywhere?
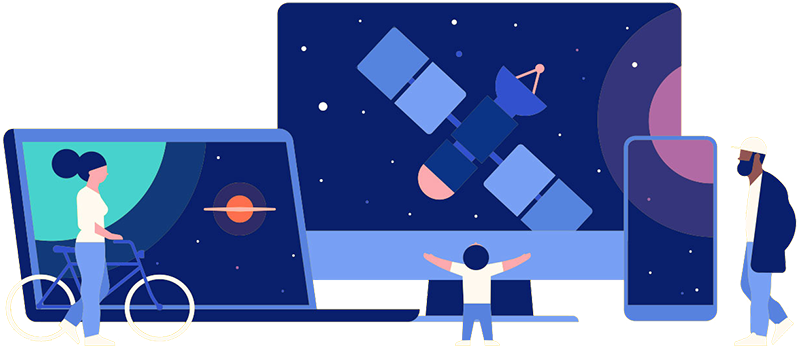
Online Access | Platform & Device Access | Cable or Satellite Access | Over-The-Air Access
Visit Access Guide
Need help accessing PBS Wisconsin anywhere?
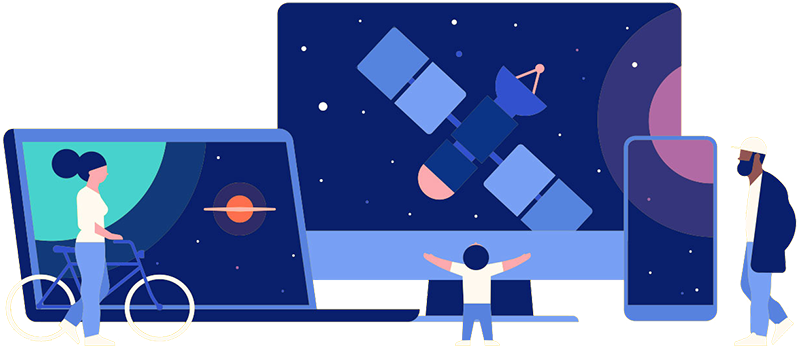
Visit Our
Live TV Access Guide
Online AccessPlatform & Device Access
Cable or Satellite Access
Over-The-Air Access
Visit Access Guide
Follow Us