[Jim Lattis, Director, Space Place, University of Wisconisin-Madison]
Well, good evening and welcome to UW Space Place. Tonight is one of our guest speaker nights. And I’m pleased tonight to introduce Professor Patrick Brady from UW-Milwaukee, who is part of the L.I.G.O. Scientific Collaboration and part of the team that made this – the really exciting first detections of gravitational waves in the past year. And so, he’s going to tell us about that tonight.
Professor Brady had a very indirect route in ending up in Milwaukee. He started, is originally from Ireland and had several positions, most recently at UC-Santa Barbara before coming to UW-Milwaukee and joining us here in Wisconsin.
So, I hope we’ll hear an interesting talk about gravitational waves and welcome Professor Brady.
[applause]
[Patrick Brady, Professor, Physics Department, University of Wisconsin-Milwaukee]
Thanks, Jim.
I – Can you hear me okay?
[Jim Lattis]
Yeah.
[Patrick Brady]
Great. Okay, so tonight I’m going to tell you about the discovery of gravitational waves that was announced earlier in the year, and to get started on this, I’m going to cut straight to the point and show you the result of a long, century-long quest. From a galaxy far, far away. . .
[laughter]
L.I.G.O. kingdom.
[static]
[static rumbles]
Now, the sounds you heard there are just a quarter of a second of L.I.G.O. data that was taken around 3:50 local time in Milwaukee, or Madison for that matter, on September 14, 2015. It’s the sound of the gravitational waves from the pair of black holes that collided in the universe 1.3 billion years ago.
Now, to put that in perspective, the first thing that I want you to think back to is imagine the Earth when it was just sort of forming and coming into being, and around 800 million years ago, the first multicellular organisms formed here on Earth.
So, I like to think about this a little bit of a race. Those two black holes collided. The gravitational waves were emitted and traveled at the speed of light away from the black holes, spreading out into the universe. And part of those waves were traveling towards us. And it was a race between human evolution to get ready to measure the amounts on September 14th. And the waves coming along. Okay?
And so, if we keep on thinking about this, the next sort of milestone that I like to put out there is the Virgo cluster. So, the Virgo cluster is a cluster of galaxies. It’s about 65 million light years away. And when you think about that and put it in perspective, that was when the dinosaurs became extinct. Okay?
And if we keep on going, the first human life formed here on- well, not first human life but the first homo sapiens, modern human beings, formed around 200,000 years ago. Okay?
And that wave is still traveling towards us. And about 50,000 years ago, the wave just crosses the outer edges of the Milky Way. Okay?
And so, it just keeps on going, traveling along through it, and we get to somewhere around 400 years ago. And at that point, it’s about as far away from us here on Earth as the north star is, as the star Polaris. Okay? 400 years. Okay?
That’s approximately the same time when Isaac Newton was born.
So, Isaac Newton was the person who came along and gave us our first real Theory of Gravitation. You know, the effect that basically keeps us on the Earth. Keeps the moon orbiting around the Earth. Keeps the Sun going around. Pardon me, the Earth going around the Sun.
[laughter]
And the Sun going around the center of the galaxy.
But then, we keep on going, and about a hundred years ago the – the – the gravitational wave is passing around the same distance as a star called New Draconis. Okay? That is a fairly dim star actually in the constellation Draco, which is the dragon. Okay?
And at that point, Albert Einstein is on the scene, and Albert Einstein comes along in 1915. And he tells us the following: He says: Look, I know Newton was great, but he didn’t get it quite right. There’s this new theory. It’s called General Relativity. And you have to think about things completely differently. You have to think about the universe as a Spacetime, and that Spacetime is curved. And all of the matter in the universe causes that curvature that appears.
And so, what we’ve come to do with Einstein’s theory is think about his equations as having two sides. On one side is the Spacetime curvature, on the other side is the matter that’s in the universe, and then the interplay between the two is that Spacetime tells matter how to move through its curvature and matter tells space time how to curve. And that interplay is the thing that Einstein’s equations told us about.
So, whenever you have matter, it curves out the Spacetime, and then something moving through the Spacetime tries to travel along what we called the shortest path in Spacetime. The technical term is geodesic, but that’s not so important. Okay?
So, Einstein did this in 1915. But, you know theories come and go. Lots of people have great ideas and great theories. So, why did Einstein know he was onto a good thing?
Well, there had been these observations that were made a long time before, in the mid-1800s, and Levarie had actually taken a look at them. They were observations of what’s called the transit of the planet Mercury across the front of the Sun. So, on May 9th this year, there was a transit of the planet Mercury that was observable. And what this image here shows you is the little dot represents – is actually the planet Mercury, okay, with the Sun behind it.
And over the course of a number of hours when you’re observing from the Earth, the planet Mercury comes across the face of the Sun and goes out the other side.
And so, you can measure the time when it starts to cross the sun and the time when it exits on the far side. And you can record that over many, many years. And Levarie took those numbers, put them together, and used Newton’s theory to try to explain how often and exactly when those transits should occur. And he found it was a problem. It didn’t quite work. Okay?
Now, Newton’s theory tells us the following: When we look at it, it says the Sun is this thing that we’re considering at the center of our solar system, and planets orbit on elliptical orbits around the Sun. And that’s represented by this orange dot with a blue orbit going around it. And that’s meant to be the orbit of Mercury according to Newton’s theory.
But the change and the difference between Newton’s prediction for the transit and the – and the observation for the transit actually gets accounted for by Einstein’s theory. And in 1915, the first thing he did when he went and built his theory was checked this. And he was able to show that these orbits don’t actually sit on that constant ellipse, like I showed initially from Newton’s theory, but they actually precess a little bit. Not shown by the lightly shaded orbits as they go around, in this case. And that exactly matched the observations. And so, for the first time there was an explanation for that problem with the timing of the transits of Mercury. And you’re going to see in a moment, repeatedly, that means that this was explained. Okay?
Now, the second thing that Einstein did – he had a few hints from his earlier – earlier work on Special Relativity – he went and did a calculation that showed that light should actually be bent by massive objects. And so, in particular, if you have the Sun and you have a star that you would think is hidden behind it – that’s where the real star is on this image – when you look at the Sun it appears to be shifted. It appears as if the star is actually, sort of, slightly away from where it really is. And that’s caused by the bending of light as it passes by the sun.
Now, in 1919, Eddington led an expedition which went down to observe a solar eclipse, and using the solar eclipse, they could look for stars that were very, very close to the edge of the Sun where the light was coming from very, very close by, and by doing that, they were able to then measure the bending of light.
They didn’t do great, but the experiment was good enough that it basically launched Einstein as this rock star of modern science, having predicted that light actually bends in a gravitational field. And I always like to show the following image, which is a Hubble Space Telescope image, in which down on the bottom right-hand corner of it, you see some object, which is actually a galaxy, which is fairly bright, and around the edges of it you see a ring called an Einstein ring.
And what’s basically happening there is a galaxy in the distance is emitting light, and that light is passing by a really big galaxy between us and the distant galaxy, and it causes the light to be bent into a ring. Okay?
And this is a gorgeous observation and a gorgeous confirmation of Einstein’s bending of light. So, once again, confirmed.
[laughter]
Now, the next thing that Einstein noticed, he’d actually again sort of figured this out from his – his thinking about Special Relativity a number of years before. He had surmised that in a relativistic Theory of Gravity, clocks should somehow run differently at different altitudes above the Earth. And so, I’ve got two, sort of, symbolic clocks here on my diagram with the Earth. One is up in the air, the other is on the surface of the Earth. They’re showing the same time at the moment, but Einstein was able to show that as they ticked by, they ticked at slightly different rates. The lower one, the one that’s closer to the Earth, ticks slightly slower. And so, what you have is that the upper one is slightly ahead. And he predicted that initially in 1907, but he made a calculation of how much that difference should be in 1915. And then the first measurement of it came in 1960 by Pound and Rebka. And I love this photo – this sequence of photos also because it just reminds you of how far we’ve come. They’re at the top and bottom of a tower in Harvard University with phones. You know, who would be doing that these days? We’d be using our cellphones, of course. But in 1960, Pound and Rebka came in and they confirmed that. Okay?
So, these are three big confirmations that Einstein’s theory is correct. Now, right away after Einstein had actually written his theory down and given his presentation to the Prussian Academy, there was lots of interest in it. And there was this other scientist who was actually working on the – the – the Eastern Front. And because this was during the war, the First World War. And his name was Karl Schwarzschild, and he said, well, Einstein’s told me these equations so I’m going to sort of play with them.
And he played around with them, and he found what we call in the field an exact solution of those equations. It was named after him. It is called the Schwarzschild Solution. And the – the – you’ll notice I’ve got a – Ive got a slight typo there. It says 2015. Well, that’s going to be important later.
But he – he predicted this solution. And when he had it, this has become known as a black hole. Okay?
So, in 1915, Schwarzschild had actually found this exact solution of Einstein’s equations that represents a black hole. People didn’t understand it, and people didn’t understand it until the ’60s. And I’m going to come back to that as we go through this talk.
So, going back to Einstein, however. Einstein didn’t sit on his laurels. He kept on going, and in 1916 he actually wrote down a set of solutions of his equations also. And these solutions were very special. They basically showed that his Theory of Gravity allowed for waves of gravity. Waves in the gravitational field.
And when he studied this, what he showed was that if you get a lot of matter. So, I’m going to get a lot of matter. My fist. Okay? And you pull it together and you make it kind of lumpy, alright? And then you shake it around as fast as you can. Okay? Now, as I do this, gravitational waves are traveling away from me, through you, through the back of the room, out into the universe at the speed of light. Okay?
So, to get a gravitational wave, he said: Get a lot of matter, make it lumpy, and shake it as violently as you possibly can. Alright?
So that was in 1916, only a few months after he first published his theory. And the – we’re going to see in a little while that he was also, as time passed, able to work out what might actually produce those things in the universe.
But, interestingly enough, Einstein, when he wrote this solution down, he said: You know, this is going to be really, really small. And we’re not going to be able to ever measure it. It’s really just a toy problem. You’ll never be able to measure it. Another demonstration of just how far we have come in the past hundred years or so.
So, what’s the effect of a gravitational wave? I’ve told you that if I get some matter and I shake it, okay, that it generates the wave. But what happens if a wave, instead of just the one I just caused, what happens if a wave comes from somewhere out in space and travels through the room?
So, I’m going to imagine this wave coming from the back of the room and traveling through and through back through the plane of the screen here and out the back of the room again. And gravitational waves can travel through material. Basically, they don’t get affected at all by the matter around us. Alright?
So, to sort of visualize the effect, we’ve got a picture of me. It is a younger me. And, as you can see, that’s a nice picture. It’s got a circular form, okay? Undistorted. Well, as a gravitational wave passes, what it does is it stretches and squeezes Spacetime, causing the curvature to change a little bit, to ripple as the gravitational wave goes by.
And that effect stretches and squeezes in perpendicular directions. And so what I’m going to show you in the next animation, which was done by Jolien Creighton, is how it stretches first in the vertical, squeezing simultaneously in the horizontal, it goes through a cyc – half a cycle like that, then goes back to normal, and then it stretches in the horizontal and squeezes in the vertical, and then comes back to undistorted again. And that repeats as the waves roll through.
So, think about it as waves on – on – on the lake or on the sea. And as they roll through, but as the gravitational waves roll through, this stretching and squeezing effect is going to continue. And so, this animation just demonstrates it.
So, the blue is the circle. You notice that there’s a red distortion that goes to an elliptical shape with stretching and squeezing occurring for the second phase. Then back to a circle, and then the stretching and squeezing is in the other direction. I’m going to play that one more time. So, let me do it again.
So here we go.
So, watch the red on the other side. And then it all fades and comes back to this. So, midway through, you’ve got the blue, which is the original circle, and you’ve got the distorted shape of me – right? – as we go.
We’re going to talk about how strong a gravitational wave is by asking how different the distance is from the center of the blue circle to the edge of the red ellipse there and by comparing the two lengths.
So, what we’re going to talk about is this thing, which we call the distortion, okay?
This funny symbol, delta L, is the slight change in length because of the stretching in the vertical direction. The original radius of the circle is L. And if you divide one by the other, you get this distortion, this strain. And we refer to it as the gravitational wave strain. Okay?
Now, it turns out that the gravitational wave strain caused by a gravitational wave from something like about the mass of the Sun shaking around at roughly the speed of – of light, somewhere out in the universe around, sort of, 300,000 or so light years away, okay? Would generate gravitational wave that is around delta L over L, or strain of 10 to the power of minus 21.
Now, 10 to minus 21 is a smallish number for those who remember how the powers of 10 work. If you don’t remember how powers of 10 work, here we go. So, you’ve got 21 zeros, followed by one. Okay? Alright?
So, there’s 10 to the minus 21. And that, by itself, of course, okay, that’s small, but, really, what exactly is that? Well, let’s – lets just try and put it in perspective by actually taking a couple of lengths, one at least that we can understand. So, the width of a human hair. Alright? So, let’s make delta L the width of a human hair, and figure out what we’d have to compare it to in order to get 10 to minus 21. Well, the answer is the distance to Alpha Centauri. Which is the next nearest star to us, which is 4.2 or so light years away. Okay?
So, that’s the type of strain that is caused by these gravitational waves passing the Earth. And, of course, at this point now our all understand why Einstein thought we’re not going to measure those things ever. Okay?
But nature has got some pretty cool recipes for making gravitational waves. And the one I’m going to focus on today is the one that we’re going to end up being the one that was the first detection. It is a pair of black holes that are going around each other, and the basic concept here is a black hole is a very massive object. So, you somehow have got a lot of matter together. You’re going to get a pair of them, so you’re going to have two things which have a lot of matter. Okay?
So, you’ve got two lumps, and they’re going to go around each other very, very quickly. And as they do, because they’re going around like this, you’re shaking the matter violently, and as they do, they generate gravitational waves.
So, here’s a – a simulation. This actually is true to scale. There’s a whole bunch of scalings going on to this. But this is simulation done by the Simulating eXtreme Spacetime Collaboration. It shows what represents a couple of black holes. So, you notice that one seems to be bigger than the other, and that truly represents mass on both of them. And the red streaks here represent the directions, the paths over which they’re moving. Okay?
Now, they’re orbiting around each other just like a planet orbits around the Sun in our solar system, except you’ve got a pair of black holes doing this. Alright?
So, I’m going to just play this through. It lasts about a minute. So, it’s a little bit slow, but I’ll say a few things as we go.
So, first up, you see these things orbiting. Now, because they’re going around like this, they’re generating gravitational waves, and those gravitational waves are spreading out into the universe at the speed of light. As they do, that amount of energy that’s leaving the system has to be compensated somehow, because there is conservation of energy as far as we know in this universe. And what happen is the two black holes get closer and closer together, spiraling into each other, until, wham! They – they collide. Alright?
And we know this because of Einstein’s equations. We can actually calculate exactly what a pair of black holes like this should do. We can put them in orbit. We can use supercomputers to simulate them. And that’s what the SXS collaboration did in order to get this simulation, which is, as I say, scaled to represent what would happen with a pair of black holes. And we’ll come back to which pair of black holes these are in a little while.
So, that gets us to the place where we’ve arrived at the, sort of, basic concepts that there’s this prediction. We know how gravitational waves are generated. We have a recipe for them in nature. But how do we go about measuring them? And this is a time where I’m going to focus in on the one type of measurement that succeeded, and that’s the one that’s based on laser interferometers. And on this next slide I have a number of different things that you can see.
The first is a picture on the top right. That’s a – a professor, an emeritus professor now, from MIT. The picture dates back to around 1972 when Rai Weiss became interested in detecting gravitational waves. And just to give you a sense, in around that time, okay, the gravitational wave that we heard on that very first slide was passing by the star Deneb, which is about the 19th brightest star on the sky. It’s Alpha Cygni, which means that it’s the brightest star in the constellation Cygnus. And it’s the head of the Northern Cross. If you’ve ever actually tried to look for the various asterisms, it’s the head of the Northern Cross.
So, the gravitational wave is speeding along and is about as far away as -as Deneb. Rai Weiss says, basically as a result of actually setting a tough homework for problem for his students at MIT, he says: You know, I wonder if I can measure these things. And he sets about it and he starts to write a whole – a whole description of how you might build a detector using this newly found technology called a laser. Okay?
We’re all very familiar with lasers these days. We take them completely for granted. We’re all aware that they can help measure distances because we know they get used for all sorts of ranging and ranging things. But he said: Well, you know, maybe I can use this to somehow measure these strains from gravitational waves passing. And the way he did it was to set up an instrument, and he – he taught through the engineering of the instrument. And the instrument would have a laser, and the laser is this cylinder down the bottom left. That – that laser would shine onto what’s called a beam splitter. So, it would split the laser beam in half and send it along perpendicular directions down what we now days refer to as the arms of this interferometer. It would go all the way down to where the black dots are, and those represent mirrors in this interferometer. The laser beams would bounce off those mirrors, come back towards the middle, and at the beam splitter again they’d get recombined. And just a little bit of light or dark, depending on exactly how things are set up, would get sent off onto that square on the right, which is a very schematic representation of a thing called a photo detector.
Now, photo detectors, if you don’t actually, sort of, can’t place them at the moment, most people at some point have driven in or out of a garage where there is – they – they have an automatic garage door. And when you drive through the garage door, you know, and the door is coming down, then by interrupting a slight beam of light across it, you actually stop the garage door, it goes back up, and you have a situation where you don’t get crushed by the garage door.
Well, the point is that’s a beam of light that’s sent from one side to the other side where there’s a photo detector picking it up. Okay?
So, this is something you do also experience in – in everyday life.
So, if I play this, it’s going to show you what happens to the laser, and then you’ll also notice as it goes through it’ll simulate the effect of a gravitational wave coming from directly overhead of this piece of – this – this instrument where it passes down through the instrument and it causes the instrument to – to shake. Because you can imagine that the beam splitter is at the center of the circle, the mirrors are at right angles along edges of the circle, and so if a gravitational wave passes by, it does this type of thing here, squeezing and stretching. Okay?
So, let’s watch what happens. So, the laser goes out, gets split, bounces and comes back. It set up initially in its perfect circle. So, no light lands on the photo diode. When a gravitational wave passes, it shakes the mirrors and causes the light that comes back to interfere in a different way than when the instrument was set up with exactly the same arm lengths initially. And this represents destructive interference. So, you get no light arriving at the photo diode. But when the gravitational wave passes, it causes this difference in the interference pattern, and so you get light and dark arriving on the photo diode. And this is the basic principle that underlies the Laser Interferometer Gravitational-Wave Observatory. And it was first written down in 1972 by – by Rai Weiss.
Now, he wasn’t the first person to actually think about the possibility of using lasers to make these measurements, but he was the first person to do a detailed design study. And so that’s why he gets so much of this credit because he actually really went through what you would have to do in order to achieve the type of sensitivity that we knew we needed being around 10 to the minus 21.
So, as this continued, over the next few years, Rai got together with a number of other scientists, and in particular a group of three known as the Troika these days. They got together, and they were scientists at Caltech and MIT. Ron Drever and Kip Thorne were at Caltech, and Rai Weiss was – was at at MIT. And they got together around the late ’70s or early ’80s and began to plan to build a gravitational-wave detector that would actually be capable of measuring gravitational-waves.
Now, the thing about this is that I want to again remind you of sort of the scale here. So, the first thing is there’s a lot going on in this diagram. On the left, you’ve got a diagram which is a schematic diagram of L.I.G.O., the Laser Interferometer Gravitational-Wave Observatory. There’s a few other things going on there, but I’ll come back to them in a moment. On the right-hand side, you have what is the ultimate thing that they built, which are two detectors. The one on the top end is L.I.G.O. Hanford. It’s in Washington state. And the one underneath is L.I.G.O. Livingston. It’s in Louisiana.
Now, these things are huge. If you look over here at the diagram on the left, you’ll notice that you’ve got your laser. The little dark on the – on the far left of the diagram. You’ve got a beam splitter, which is shown as the little angled piece of – of glass that’s indicated in there. You’ve got a few other things going on. It’s a little more sophisticated than the previous cartoon, but you get the basic idea. Down the end of each of these perpendicular directions there is a thing referred to as a test mass, though they are actually mirrors. And the length of each of these arms is four kilometers. Alright?
So, these are big. Alright?
The mirrors that are hung at the ends of these arms are about 40 kilograms. That’s about 80 pounds each. Alright? And if you want to, sort of, figure out the 10 to the minus 21 number and ask how much, how many centimeters does one of these mirrors have to rattle around in order to get you 10 to the minus 21 when you compare that little rattling to four kilometers? The answer is 10 to the minus 17 centimeters. And, again, 10 to the minus 17 doesn’t really mean a lot, but if you think about this and go through and read some quantum mechanics books and a few other things, what you find is that’s about one ten-thousandth of the size of an atomic nucleus. Now, atomic nucleus is pretty darn small. And that’s a pretty amazing feat. These instruments can measure a change in position, okay, that is equivalent to one ten-thousandth of an atomic nucleus.
Now, I’ll come back to that a little while later, but for now we should actually, I – I want to just continue to move on to mention that there’s a number of things about this experiment. There’s a whole bunch of tech-technological challenges. One of the first things is that in order to do this measurement, you’re using the wavelength of the light as a ruler. Okay?
It basically is the thing that allows you to measure distances. You do everything compared to the wavelength of the light. And so, in order to be able to do this, you need to be able to have a laser that can be very powerful. And if you take a look here, the power of this laser going in is 20 watts. That’s actually not terribly powerful by modern standards, but the requirements on the stability of the wavelength are extremely stringent And so, it’s very difficult to get high power alongside tight and very precise wavelength measurements.
Also, when you start to actually build the instrument, you figure out that there’s a few other things that go on. So, for example, if I jump up and down here, you can probably feel it in the back of the room almost. Okay? And the bottom line is that when the Earth – when the Earth has stuff rattling up and down on it, the Earth vibrates, and, of course, that’s going to shake anything that you build and put on the Earth. Okay?
That seismic activity of any kind will actually shake this instrument, and in particular, that seismic activity is just going to cause these lengths to change in ways that might actually mimic gravitational waves. This limits the frequency band where we can listen for a gravitational wave. We typically cannot hear gravitational waves with frequencies that are less than around 10-20 Hertz. Okay?
Now, to put that in a context that you can kind of think of, if you think, roughly speaking, 100 Hertz is a good sort of frequency range in which to think about what you can hear, okay? It’s just roughly audio for human beings. Alright? So, it turns out that these instruments, as a result of the technological challenges, are sensitive to gravitational waves that come in and vibrate about 100 Hertz. That’s 100 times per second those gravitational waves stretch and squeeze Spacetime as they pass by. Okay?
That’s the frequency range that we’re most sensitive to. And we’re going to come back to that in a little while.
So, with that in mind, let me also mention the next thing. I’ve already told you that each one of these instruments is four kilometers long, so it’s pretty obvious that L.I..G.O is big science. But I like to kind of give a sense of what it means to do big science and how long it took us to get from 1972 to 2015, right? And to go from a concept to an actual measurement of a gravitational wave.
So, if we think about the time scale, you’ve got the feasibility studies in the ’70s, you’ve got the first funding for construction in 1992, you’ve got first light coming in around 2002, and then we went through a set of observing runs where we tried to find gravitational waves and we didn’t. We didn’t succeed. And at the end of that, which was around 2010, we took out the initial instrumentation and did a – did a large upgrade on the instrument. So that was around 2010.
So, let’s actually think about that for a moment. Where was this gravitational wave in 2010, okay? So, that gravitational wave in 2010 was passing somewhere around the same distance as Alpha Centauri, right? Somewhere around five light years away, four or five light years away. So, it was just coming to the place where it was passing by our next nearest star, and there were no stars between us and there, right? And so, in 2010, of course, we’re just like: Yeah, tear out the instrument, break it down, right? So, we didn’t realize that this wave was coming in and still we’re in a race to try and get there.
But 43 years after Rai Weiss first wrote his note, we ended up with this beautiful observation of the data of a gravitational wave signal coming from a pair of colliding black holes 1.3 million light years out in the universe. That means that they collided 1.3 billion years ago, and the waves traveled to us over that entire period of time.
And so, we’re back now to the beginning of my story. And I’m going to play for you again the sound you heard at the start, but I’m going to reference a little bit more about the sound that you hear. As I said already, the sensitivity for the – that we have with the L.I.G.O. instruments for gravitational waves is that we’re sensitive to gravitational waves that are around 100 Hertz, that’s around the audio frequencies, and when I play this, I play it in two different ways. I play the original data, that’s the first time you hear it, and then you hear it modified a little bit. The second modification is simply to try and bring the sound out a little bit, make it a little easier to hear. So, we adjust the frequencies a little bit. But the first is basically the frequency of the gravitational wave as it passed the Earth. It lasts about a quarter of a second. And, again.
[static rumbles]
So, for me, that’s the most beautiful sound in the universe.
[laughter]
And – and on September 14th, 3:50 local time, I can tell you I was asleep. We were not actually set up to go on a full-scale observing run at that point. We had started about three days before with what we call engineering time. That’s a time when we basically get out our wrenches and we start tightening the bolts on the instrument, we start making sure our data analysis on computers are all working. We get all of our stuff ready to go, but nature just said: Hey, you know what, here you go. Two black holes colliding, get on with it. And so, we did.
And the timeline, the timeline for this discovery is something that, for me, was just fantastic. This was the culmination for me of 20 years of involvement with – with gravitational wave searches. Of course, it was the culmination of 43 years of involvement with gravitational wave searches for Rai. But, nevertheless, it was still fantastic. And the – the- the timing over which this discovery unfolded was also just really impressive.
So, within 10 seconds of the data arriving at the two instruments, it had been calibrated. That’s our way from taking the electrical signals that we record and turning them into this thing, this strain measurement that I mentioned. And we take that calibrated data and we ship it from those two observe-observatories to Caltech, where we then have a set of data analysis codes which crunch through that data. There’s – theres a huge supercomputer there that crunches through the data and looks for possible signals in the data stream.
Within three minutes, we had what we call a trigger submitted to the Gravitational Wave Candidate Event Database. That is something that’s hosted in Milwaukee on UW campus. And it came with what we call a false alarm rate of once per 30 years. What that means is that if this wasn’t a gravitational wave, we’d expect that somehow a rattling of the Earth or some other instrumental noise would cause this about once every 30 years. So, it was very rare to have this happen from noise only. So, this immediately alerted us to the fact that this is interesting, that once per 30 years. Within an hour, the collaboration had actually started to wake up, people were being called. Get out of bed, start, get on with the job here. We need to freeze the instruments. Don’t change them. Let’s make sure everything is okay because one of the things we were very concerned about with this measurement is it’s incredibly precise. I’ve already told you, one ten-thousandth of the size of an atom is the type of measurement we’re talking about. So, to convince the world that it was real, we needed to make sure that there was nothing wrong with the instrument that had actually caused this signal to be there. So people went around that day with cameras photographing every single place on the instrument they could so that they could go back and check that no one had put any sort of bad – bad piece of instrumentation in there, nothing was missing, nothing was broken and other things like that.
So, we had a huge effort ongoing within hours of the – of the gravitational wave passing the Earth.
Within a couple of days, we knew that it was a signal, a gravitational wave from a pair of black holes. We could figure out how big those black holes were. We knew they were approximately 2029 times the mass of the Sun and 36 times the mass of the Sun. We knew that they had collided about 1.3 billion light years away. So, that had happened that the collision itself had happened 1.3 billion years ago. And within a couple of days, we alerted other astronomers that we partner with to go and look in the direction that we thought the gravitational wave came from to see if they saw anything with a regular telescope, with a standard telescope.
Now, one of the things that is actually fascinating about this discovery is the interplay between the original theory, Einstein’s theory, and the observation itself. Without actually having both sides of this, we couldn’t know much about what the gravitational wave was that we – that we came across. And so, to, sort of, demonstrate this, I – I – I do this simulation. So here, again, you’ve got – this is a graph made of our data. Its strain is what’s represented by the various curves. You’re going to hear the sounds in a few moments again. And I’ve done – Ive indicated what we call GW150914. That’s 2015, September 14th. So that’s the gravitational wave that we saw. As you can see, you see a little sort of spike that looks a bit bigger than the rest of the data. But off to the right, I’ve added – Ive added something else into the data stream just to sort of give you a comparison of a couple of different sounds and to try, in a few moments, to bring out how it is we know it’s a pair of black holes.
So, let’s play this for a moment.
[static rumbles]
Alright? So, as you can tell, two different sounds. Now, clearly, to your ear, you immediately hear they’re different. It’s just completely obvious. But how do we know what one or the other is? Well, in the case of GW150914, we compare that sound with predictions computed using numerical solutions of Einstein’s equations.
They’re done on big supercomputers, and we simulate pairs of black holes going around each other and colliding, and then we use a bunch of really hard calculations to try and make – to try and figure out other aspects of that, and we stitch it all together, and that tells us what the gravitational wave from a pair of black holes that are 29 solar masses and 36 solar masses colliding with each other should be like.
And this simulation on the next slide, which actually, that’s a little hard to see. Let your eyes adjust a little bit. You’ll see two dark black dots. You’ll also see some green shading. As it goes through, it gets brighter. So, youll – you’ll see it as it goes. But as we go through, you’ll notice that the black dots are going around each other. And they represent the event horizons of the two black holes. The black holes are orbiting each other. They’re generating gravitational waves. The gravitational waves are represented by the bright green spreading outwards from the orbiting black holes. The orbiting black holes are getting closer and closer together until they collide and turn into a single black hole, at which point the gravitational wave emission stops.
They settle down to a single black hole, no more gravitational waves being emitted.
So, when we make that calculation, we can figure out what the sound should be like at the instruments here on Earth. And we can compare that sound that we predict with the sound that we measure. And here, up on the top, is the data that we measured at both L.I.G.O. Hanford and at L.I.G.O. Livingston. And down at the bottom is the calculation of what that gravitational wave should be coming from a pair of black holes that collide out in the universe.
Without the simulations, without the hard calculations, without big computer programs to compare those calculations with the data, we could never, ever pull this out. Okay?
So, this is a – is – is a place where all sorts of technologies merge. We get big computing technologies. We get the theoretical technologies associated with Einstein equations. We get the modern technologies, like lasers and other things, all coming together to allow us to do gravitational wave astronomy.
So, let me come back for a moment to this.
So, what on Earth did I add into the data? Let’s listen to it again. You can form your own opinion.
[static rumbles]
And the answer is I added in a bunch of people.
[laughter]
This was a – a public talk that our students and postdocs gave in Milwaukee about a week or two after the announcement. And what – one of the things that was going on at the time was we used to ask the audience to actually try and generate a chirp, the wave form that you hear. And that was their attempt.
[Woman, off camera on video]
Again. One, two, three.
[group on video chirps]
[Woman, off camera on video]
Thank you so much. You were great.
[applause on video]
[Patrick Brady]
So, as you can tell, by listening to the structure of the sound, you can actually tell information about what generated the gravitational wave or what didn’t if it wasn’t a gravitational wave. And that’s important for us too.
So, at this point, I’ve actually got you to within a few days of the gravitational wave passing the Earth. And, from there, we actually had to get hard to work and we set to work for quite a while. One of the most important things we had to do was demonstrate that this was a very rare thing to happen due to noise only. And in modern physics, the typical way that this is stated, doesn’t have a lot of meaning by itself, is that the discovery of is greater than five sigma. Now, what does that basically mean? Well, what it means is that if this was caused by noise of some kind coming from the instrument, okay? it was more rare than once per 10,000 years. Okay?
So, we would not see something like this, okay, more often than once per 10,000 years. And in actual fact, it’s far less than that. We actually think it’s way down at one in a million years, but we were only able to bound it. Okay?
We knew this at six weeks afterward, but we still had a lot of work to do. And over the following five months, three months roughly after that, we had to just check, check, check. There had been too many, really, discoveries announced in recent years where scientists quite – hadn’t quite pulled it together quite well enough. There had even been a tentative announcement of gravitational waves the year before by a different experiment through a totally different methodology that turned out to be wrong. So, we just kept on checking. And we got to the point, after about five months, that we were able to write a discovery paper. We sent it off to the journal, and it went through the academic process of peer review before we told anybody in the news media or anywhere else that we had made this discovery. We had about 11 other papers that we had to write. There’s a sequence of them right there. And many of them were ground – ground-breaking. I’ll explain in a little while just how ground-breaking this is in some respects.
But wait. Somewhere in the middle of that, I was back in Ireland. It was Christmastime, and I went to sleep on – on Christmas night. And at around 3:38 in Europe. I really think gravitational waves have it in for me. I was still in bed, but another gravitational wave that was measurable by the L.I.G.O. instruments passed by and was identified. Within a minute, we had a trigger up in the Gravitational Wave Candidate Event Database again. The false alarm rate for this was once per thousand years, which was even more rare than the original alert for the one that we saw in September. And so, we knew the game was on again. We had a second candidate that was probably a gravitational wave. And it turned out to be – to match the signal we expected from a pair of black holes 14 times the mass of the Sun and eight times the mass of the Sun colliding with each other about a billion years ago. Alright?
To give you a sense of this, I want to just play a couple more things. So, this here is going to be.
[rumbles]
So, this is a natural pitch. The first sound.
[rumbles]
That’s the one on December 26th.
[rumbles in succession]
That’s September 15th. This is that increased pitch.
[4 chirps in succession]
So, what I really want you to take away from that is the difference in sounds. You can hear it, right? You can tell these are different. Even with your ear, you know they come from something different, okay? And that difference that you can hear is what we can check using Einstein’s equations and the supercomputers to compare the data that we measure with the simulated signals.
So, within five months, we had an announcement on the 11th of February of 2016. Gravitational waves finally directly measured and confirmed with an article published in the Physical Review Letters, and it was – it was pretty exciting. I was out in D.C. at the National Press Club, and it was – it was – it was really amazing. The interest generated by it was substantial. There’s just been a lot of – of things going on. But the thing that I want to, more or less, not quite leave you with, I’ve got one more thing after this. But I want to just highlight about all of this is to try and put in perspective what this means for astronomy.
So, this is a cartoon graphic. It’s an infographic that is trying to show something about the black holes that we know about in the universe. It’s focusing attention on black holes that are of masses that are around the same as the masses of typical stars in the universe. And, on the left, with the caption “X-ray Studies,” you’ll notice that there are these, sort of, you know, two, four, six, eight, ten, you know somewhere around 14 black holes represented. The size of the little circle represents the mass of those holes. Alright? And those black holes on the bottom left were all of the stellar mass black holes that we were pretty sure about in the universe before September last year.
After the detection on the 26th of December, we had two confirmed black holes, GW, or pairs of black holes colliding: GW150914 and GW151226.
And the interesting thing about those is that you have actually two black holes collide and form a third. So, each one of those observations represents three new black holes that we know about in the universe.
So, we added six confirmed black holes to our list of black holes that we knew about in the universe by making those two detections. It turns out we also have another interesting thing going on in our data. In – on October 12th, there is something interesting there. We’re not willing to call it a guaranteed detection of a pair of black holes, but it looks awfully like it. So, we’re pretty sure that what we’ve actually done is we’ve added nine new black holes to the inventory of black holes we know in the universe. And that’s with only looking at the universe and gravitational waves for about four months. Okay?
So, where do we go from here? This is my second from last slide. I’m just going to go through these very quickly. The first is that the future of gravitational wave astronomy is now. We have the two detectors in North America, the one in Hanford and the one in Livingston. We have got partners in Europe. There’s a detector called Virgo that is in Italy, and that’s a large detector that should come online sometime next year. There’s a smaller dectect-detector, GEO. It’s only 600 meters. It’s not the four kilometers long on a side. So, it’s not as sensitive, but it’s part of our network. And we’re going to add two more detectors over the next decade. There’s going to be the KAGRA detector in Japan, and there’s going to be a new L.I.G.O. detector in India. The location for that detector was just selected over the last month or so.
So this is the future, and by 2023 or 2024, we’ll be observing the universe using gravitational wave detectors distributed around the world, and the more of them we get in play, the more information we can extract from that signal, the more we can learn about the objects we’re observing.
But it’s not the only story. The truth is that there are other ways to look for gravitational waves. We could put detectors up in space. The basic principle is the same as the Earth-based ones. You use lasers to measure the distances between mirrors. But the plans for these typically involve putting spacecraft up in space where they’re actually about a million miles apart, okay?
So, it’s kind of complicated. Right now, European – the European Space Agency is planning a launch date of 2034 for what is called eLISA. eLISA, or some variant of it, has always been launching somewhere around 20 years in the future for me. So, we’ll see if that happens. There’s another method that’s in play, which is the International Pulsar Timing Array, and the North American Nanohertz Gravitational Wave Detectors, and that’s called NANOGrav. They use pulsars in the universe to try and detect gravitational waves. And each of these are sensitive to gravitational waves at very low frequencies. So, they’re not in this hundred Hertz audio region. They’re way, way down at much lower frequencies.
And so, with that, I’m going to finish and say thank you.
[applause]
Search University Place Episodes
Related Stories from PBS Wisconsin's Blog

Donate to sign up. Activate and sign in to Passport. It's that easy to help PBS Wisconsin serve your community through media that educates, inspires, and entertains.
Make your membership gift today
Only for new users: Activate Passport using your code or email address
Already a member?
Look up my account
Need some help? Go to FAQ or visit PBS Passport Help
Need help accessing PBS Wisconsin anywhere?
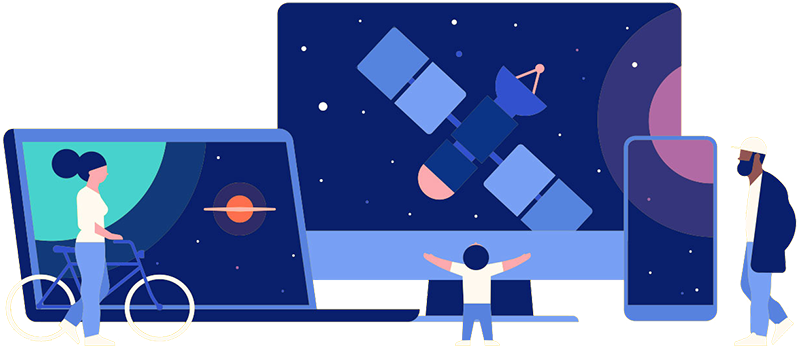
Online Access | Platform & Device Access | Cable or Satellite Access | Over-The-Air Access
Visit Access Guide
Need help accessing PBS Wisconsin anywhere?
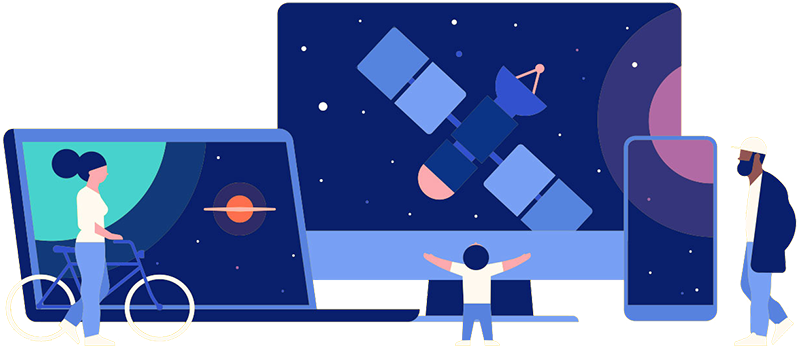
Visit Our
Live TV Access Guide
Online AccessPlatform & Device Access
Cable or Satellite Access
Over-The-Air Access
Visit Access Guide
Follow Us