– Welcome everyone to Wednesday Nite @ the Lab. I’m Tom Zinnen, I work here
at the UW-Madison Biotechnology Center. I also work for the Division of Extension and on behalf of those folks and our other core organizers, Wisconsin Public Television, the Wisconsin Alumni Association, and the UW-Madison Science Alliance,thanks again for coming to Wednesday Nite @ the Lab.
We do this every Wednesday night, 50 times a year.
Tonight it’s my pleasure to introduce you to you Elle Kielar Grevstad, she’s in the Department of Biochemistry. She was born in Woodstock, Illinois and went to high school in McHenry, Illinois. And then she came up to UW-La Crosse to double major in both biochemistry and in cellular and molecular biology. Then she came to UW-Madison to get her PhD in biochemistry, and then in 2014, she returned to UW-Madison to help launch the new Biochemistry Optical Core. Tonight she’s going to talk to us about “From Cheddar Cheese to Single Synapses: “Light Microscopy Across the Disciplines,” and I must say there are very few scientific words that rhyme with cheese the way that synapses do.(audience laughing) Way to go.
This is going to be quite the visual treat. Things have changed a lot since I first put eyeballs to a microscope back in about 1971. First of all, the world is in color. Second of all, you can record it, third of all, it moves,
and fourth of all, things can be alive. And that’s pretty astonishing things.
It’s great to see where microscopy has gone in the last 30 years or so. So please join me in welcoming Elle Kielar Grevstad to Wednesday Nite @ the Lab.
(applause)
– Can everybody hear me okay? Pretty good? Okay. Thank you all for having me.
Thank you, Tom, for the invitation to talk. We spoke in the hallway one time
and I had talked to him about how I, about our core facility, and how we get people who image cheese and then in the same day we have people who are looking at neuronal synapses. And he thought that was just fantastic and he wanted me to speak with you guys about the breadth of research that microscopy supports on UW, on the UW campus.
So the title of my talk today, like I said, is “From Cheese to Single Synapses: “Microscopy Across the Disciplines.” I’m headquartered just across Henry Mall in the Biochemistry Department and I’m the Director of the Biochemistry Core Facilities.
Just to give you guys a sort of perspective on what the Biochemistry Core Facilities mean and what it means to be a core facility, you can think of us in sort of a community lab space. People from all across the university as well as outside of the university come and access the expertise, and the instrumentation that’s in our core facility in order to do research.
There are four core facilities on camp us so I’ve just highlighted this, this is us in red, the Biochemistry Department, we’re here tonight, right? So there’s four other light microscopy core facilities on campus. The one that I’m highlighting is the Biochemistry Optical Core, and then there’s also one over at The Waisman Center that supports brain research. There are a couple over at the hospital in the WIMR. (Wisconsin Institutes for Medical Research)
And then there is also one in plant, in the Botany Department, that supports plant research.
One nice thing about the Biochemistry Optical Core is that we’re sort of centrally located. So we get lots of different types of researchers that come into our campus and come to the core facility to use us. So just for instance, right here, right by my cursor, Biochemistry’s here, Genetics is here, Metamicro’s here, Horticulture’s here, Food Science is here. So all of these people come and use us, as well as the engineers across the Great Divide of Campus Boulevard. (audience chuckling)
So in 2014, I was asked to come back to the university to start up the Biochemistry Optical Core, and our explicit mission is to provide support
and train the research community on their techniques and equipment that encompass basic to state-of-the-art light microscopy methods.
We were formally founded, like I said, in the spring of 2014, and since then
we have over 200 different researchers that we’ve interacted with, and those are from about 80 different research groups across 27 different departments on campus. And that represents about seven different colleges and schools.
You may be thinking, how does a food scientist access, use a microscope in the same way that somebody from, say, psychology, would use the microscope? And the answer is that they don’t use a microscope in the same way. But they’re all interested in the spatial orientation of molecules, or proteins, or structures in an environment.
And just to give you guys a background, I think the easiest way to wrap your head about how these multiple disciplines can leverage microscopy, I want to sort of bring us back, back in time, all the way about 350 years ago and start talking about how, the sort of invention of microscopy, and how the principles of that invention still remain the same today, as well as what has pushed it forward to make it more of a quantitative and dynamic field than it was perhaps in the early 20th century.
So, the Father of Microscopy, his name was Antonie van Leeuwenhoek. He developed these simple microscopes and for those of you who are in the audience, what you are looking at when you came in are images taken on replica microscopes. So those are, what they did is they put pond, or pond water on the specimen holder, held the microscope up to a candle, and looked at the tiny organisms within that.
What you would see if you were that person, you would see something like this. And immediately, as a scientist, and as my five-year-old daughter and my 2.5-year-old son said when looking at this, they said, “Mom, what is that? “Why are those things so small? “That’s a whole different world. “I didn’t know there were things that grew in water.” Well, my daughter’s quite bright and so she was like, “Well, why is that thing crawling on that? “How does it know to crawl on it? Right? “What are those bright dots? “Why are those organized this way? “Ooh, what is that, why is it slithering through the space and it’s not interacting with this?”
So you can imagine the sort of vast explosion of this small tiny world that van Leeuwenhoek discovered, essentially. And you can see how many questions you can ask just by seeing something.
This continued, so microscopy continued to be sort of a fad for a long period of time and this is an image, if I can stop this, which would be helpful, move forward.
So this is an image in the 1800s, the Victorians loved microscopes. In 1846, there’s these wonderful quotes by the Naturalist, Charles Kingsley, who explained, “The tiniest pieces of mold “on a decayed fruit and the tiniest animalcule,” which is tiny animal, “from a stagnant pond will imagination find “inexhaustible wonders in a fancy of fairyland.” And then in 1861, before he became the President of the Royal Microscopy Society, Henry Slack described a group of protozoa as a tree from fairyland.
We laugh, we giggle like, fairyland, that’s so crazy. Why would they call them fairies?
But if you think about this, this is 200 years ago. I sort of chuckled to myself and I think, well, 200 years from now, we’re going to be in the 23rd century, hopefully, what are the top scientists in our field going to giggle about that we thought we understood very, very well? Probably a lot (chuckles) I’m assuming.
So why is microscopy in the 1600s and the 1800s, what’s this thread, what does it, what is the connectivity? And how are the microscopes of today, what do they have in common with the microscopes of 350 years ago? And the answer is actually quite a lot. So, a modern microscope would look something like this. You would have a computer attached to it so you could digitize your images and since you wouldn’t have to be an amazing artist to be able to draw the fine synapses or structures of cheese that you were looking at. But they all have three common things, is that they magnify an image. So they make it bigger, they magnify an object. They make it bigger so that your eye can see it. They resolve details, which is different than magnification. So resolving details means that they can separate two objects in space from each other. And you can see those two objects as two distinct things, rather than one blob of a thing. And the third thing that they have in common is they send the images to either your eye or to a detector, and these detectors can be quite simple, like you’re iPhone, or they can be as fancy as a $100,000 camera.
There’s a vast variety of ways in which you can capture images on a microscope. But they all have one fatal flaw, like microscopy does. And it maybe isn’t fatal because the field is still alive and going. But they have a limitation, and that limitation is light. And it is the resolving power of light, which is what we term in our field as diffraction limit. So, if you are going to come to a microscope today, and you had an organism, anything from a mouse to a small molecule that you wanted to study, you would have to pick the type of microscope, or the type of imaging station, to fit your needs.
So anything from a mouse, you could image with a PET, or an MRI, or an ultrasound. You could even use a confocal in some, like, unique settings. If you were trying to image mouse brains or maybe an ant, or a hair, which is about as fine as your eye can see, you could bring it to a standard microscope. Once you want to start imaging single cells, or bacteria, they’re still suitable to see with a microscope because they’re right around one micron in size. But once you get below 200 nanometers, which is the diffraction limit of light, you’re no longer able to resolve individual structures from each other. So say you have two viruses that are packed within a cell and they’re close to each other, you couldn’t tell whether or not those are two viruses or one virus.
And if you were looking at the structure of proteins which are on the order of 10 nanometers, you couldn’t use light microscopy to see those individual molecules unless you did a sophisticated form of microscopy. But what you can do is you can use microscopy in order to give you spatial relationships between the organizations of proteins within cells, or the organization of viral particles within an organism, or how a bacteria moves within space.
And the reason why the diffraction limit– So I say it’s the diffraction limit of light, and I just sort of want to explain to you why it’s 200 nanometers. ‘Cause I think even in graduate school, I never really, that was thrown out there. It was oh, it’s the diffraction limit of light, it just is what it is, and I never really understood it until I had to start teaching it, like a lot of things that happen in life, right?
So, say if you want to think about why light microscopy is limited, you have to think about what does a lens do? And the lens, the thing that sees the object that you’re looking at, which is the most important part of microscope, and it is the reason why Antonie van Leeuwenhoek is considered the Father of Microscopy because he was such an artist with his milling of a lens that it allowed him to see these small animalcules that he would see. You have to think about, okay, we have one point and this point is emitting light in some way, it’s releasing light. And you’re using a lens, and this is a modern lens, it has some writing that means something to microscopists on it.
And what does the lens actually do? So, the lens is actually going to pick up the light that’s coming off of that object and it’s going to try to reconstruct the light in a particular manner. And lenses are actually not that great at doing this (chuckling) surprisingly because light acts as not only a particle, but a wave. And so, when it comes in, it’s going to bounce off the pieces of glass in the lens and interfere with each other. So what you’ll see when you’re focusing on a single point, and you focus up and down is that you’ll actually start noticing these patterns that arise. So that’s a single point, and then, right, this is a sphere, and it shouldn’t start looking like a donut. So to just to pick up where it is, is that this single point of light is, if you think of it as a ball, if you focus up and down on it, it shouldn’t ring out like this. It should stay as a single object. But because light interferes with itself in odd ways because it’s a particle and a wave, when you project what you’re seeing, what you actually see is something that looks like this, which is what we call a point spread function.
So the point is here and then the spread is what you see when you focus up and down. It’s also known as Airy disks, after a scientist who described the mathematics around this. And this is a problem because if this single point is two nanometers, this single point of light, it’s coming off an object that’s two nanometers in size, the point spread function essentially spreads the light at anything greater than 200 nanometers, which is 100 times larger than one the object actually is. And if these two points were very close to each other, you wouldn’t be able to tell one point from another. And I think that this video that I’m going to show now sort of shows how and why waves interfere with each other.
So if you look at this, if you’re looking, if you think of those two balls as a single point of light, or two single points of light, you can see the patterns right here. And then as you come out, they just look like one, does that make sense? So you have two, and then they converge, and they do constructive. They undergo constructive and destructive interference and it becomes a singular wave. And then we sort of cartoon, make a cartoon version of this, what you can see is there’s two molecules in space and they’re both going to emit light. And what happens is that these areas in which these circular waves overlap and get amplified over the background, and if you have a lens in its place, what it’s going to detect is the bright parts. And so you get this, and so it ends up looking like a single molecule in between these two objects.
And this is what we call the diffracted orders of light. So this is zero, negative one, negative two, and for those of you guys who are really interested in light you need at least to capture one diffraction order off with a zero in order to resolve objects.
So, if you think about this a little bit closely, if you have a lens, people will say, “Well, why don’t you just make a lens that’s giant “and it would capture all of those things?” Well, you could, but not really because there’s physics involved and manufacturing technology is not quite there to make a giant lens that could fit to see a two-nanometer object. But you can play along with a little bit. So, for an objective that is small, you would see this object like this, it would show up as a singular blob. If you had a larger objective, something that could collect here and here, which we have, you’re now able to start separating these objects in space.
What happens though is that when these objects become closer together, you can see that these overlapping patterns actually become tighter. And so, what happens actually is that it’s tighter but also spread out for the single order of light, so the negative one and positive one. And so you’ll see that here, and so even with the large objective, you’re still not going to be able to capture the negative one, and they blur together.
So that’s how, that’s the diffraction, that’s how I like to think about the diffraction limit of light, and that’s the limitation of microscopy. So even though these limits have always been in place, and have been known about for a long period of time, since the early 1900s and even into the 1800s, what has changed, right? Why is light microscopy nowadays so much more powerful than the light microscopy that was performed in the ’70s when Tom was in school? (chuckling) They still magnify, and they still resolve details, and they still send the images to the eye and the detector.
But what van Leeuwenhoek would be able to see, which is something like this, this gets a little muddled. This is a C. elegan, which is a worm that’s used for developmental biology to study development. You could see its little heart pumping here. But what you notice is that this would be taken on like a Victorian microscope.
And what you can see nowadays is something that’s much different. And this is the same organism.
Play?
Well… These things move around in space. (laughs) See if we can get it to go, if I can go like this. So you can see them sort of move in space and then with real time. There we go. Technical difficulties. Yeah, it’s called glonads, which is kind of funny. So this is now a CRISPR-engineered C. elegans to highlight the gonads of the C. elegan. And so the difference here was the development of fluorescence.
And what is fluorescence?
It’s the thing that provides contrast and specificity in microscopy. And for those of you guys who aren’t familiar with fluorescence, I’m just going to briefly cover it. So, it’s the game changer. (chuckles) It essentially means is that you can have a molecule which does not glow at all. It’s not like a firefly in that regards where it’s constantly admitting light. But you can hit this molecule with a certain wavelength of light, in this case, it would be green light. And then that molecule takes up that energy from the green light, and does some conformational change in its energy bonds and it will release the energy as light at a farther wavelength, usually it’s red.
So that’s just sort of depicted here. And you can see that also, in a pretty basic, you can do this at home, my kids got a kick out of it when I did it. You can take a UV light source and then put some tonic water, if you have the real tonic water with quinine in it. And you can shine light into it and the quinine absorbs the UV light and emits blue florescence. And it’s the same thing, but you know it’s clear. And what is fantastic about the fluorescence is that as soon as you shine light on it, it gives you contrast over a background. And fluorescence was actually discovered, not surprisingly, during the Victorian era in the 1800s.
These are just sort of some molecules, they’re on a whole order. A couple of these molecules, fluorescein, which is still common today, was synthesized in 1871. And these are all derivatives off of quinine, most of them. So these are chemicals that are fluorescents, but there’s lots of different ways– There’s lots of different other molecules that are also fluorescent.
And the chemists in the last 15 and 20 years have been incredibly creative in ways in which to attach fluorescent moieties to proteins, to lipids, to anything under the sun that you want to study, you can make it fluorescent and you make it any color that you want to see. Which means that you can see multiple colors simultaneously and get a relative organization of up to 20 different proteins or 20 different molecules at the same time.
The other thing that was quite revolutionary in the fluorescent field was the discovery of GFP. So for those of you guys who don’t know, GFP is taken from– It’s called green fluorescent protein. It was first discovered in the ’80s and cloned out of that organism, which is jellyfish. It’s a jellyfish protein. And then Roger Tsien, who won the Nobel Prize a few years ago for the development of all the different colors, he was able to change, just by changing the amino acid structure within the protein itself, changed the spectrum in which it gets excited and gets emitted by. And then, not surprisingly, biologists were clever again and CRISPR came along, and now you can genetically engineer anything you want, essentially, and make that protein fluorescent at endogenous levels in an organism that you’re interested in studying.
So what did it– I’ve hinted at this a couple of times. So what does it really provide us?
Fluorescence provides us a form of– So you can see this, this is a sort of flat image taken with a bright field microscope, so similar to what you would– It was a modern version of van Leeuwenhoek’s little candle. It provides you contrast and the ability to target the proteins that you’re interested in. So this is the same neuron, and now you can see the dendrites and the spines. You can see this sort of network of– I think this is actin within here, you can see the mitochondria, and you can see the nucleus all with different colors. You couldn’t see that sort of nice signal to noise over the background with just a flat image.
So this was revolutionary, right? It has been, and so how are people on campus now using these sort of revolutions? It’s not just to take pretty pictures. This is one of my pictures for those of you guys who don’t know, this is a– I love taking… Using art, or using microscopes and promoting art through it. So this is an image of a corn, of a piece of corn. It’s a seed. It’s a cross section. This is the embryo of the corn, so this is the sheath, and then this is the endosperm. This is taken on a microscope that allows you to take optical sections of this image and it’s fully spectral, which means that I can take any color on the visual light range and see it. This is great, but I mean, and its beautiful work, but it doesn’t really address scientific questions. It just sort of gets people interested in science.
So, this is maybe less interesting, but maybe a more research, maybe– It’s not less interesting at all, John would be very upset of me for saying that. This is maybe less detailed, or less beautiful, in terms of structure. John Lucey, who is the Director of the Center for Dairy Research, his group brings mozzarella to us. Really, they’ll take a chunk of cheese and they’ll slice it on our wet bench. And they’ll stain it to understand the protein structure of this cheese. And what you’re seeing here is cheese that is a week old, cheese that is three weeks old, and cheese that is six months old.
And what you may be able to tell is that the structure changes over time. And this is actually a breakdown product and so John’s really interested in trying to create manufacturing processes and natural derivatives in order to stabilize the cheese structure over time. And he uses the microscope in order to see that structure and to see whether or not his experiments are being successful and whether or not he can apply the work that he’s doing to the dairy industry.
And in another applied research setting, this is– I wish this was a little bit– This is one of my favorite images only because people get so excited when I tell ’em what it is. So this out of Daniel Noguera’s lab in Civil Environmental Engineering. Daniel’s extremely interested in trying to engineer wastewater treatment plants to be more efficient.
One thing that is one of the most costly things in wastewater treatment is the oxygenation of water, so that the microbes that scavenge the nutrients out of the water can work effectively. He is trying to engineer bacteria that function under low oxygen conditions. And so he can look at the RNA that’s getting produced in the wastewater sludge. So this is sludge from a waste, from a reactor in the wastewater treatment plant. It’s essentially a biofilm, so it’s all of the organisms that make up this sludge. And he’s staining the different genetic factors that could promote more efficient clearance of phosphorous and nitrogen out of the wastewater.
It’s pretty amazing that this is sludge and it’s so beautiful, I think, personally. I was really excited to see this. So if you start thinking about this as like these are tiny organisms, right? You may be thinking, “Oh, well, “is this really relevant into the medical world?” And I would say yes, because of people like Dr. Bruce Klein, who’s in medical and microbiology and immunology. And for those of you guys who can’t see, this scale bar is actually down here, right here, is actually 1000 microns, so one millimeter in size. This is a live rat lung, or mouse lung, sorry. So it’s a cross section, so it’s huge. This is a piece of tissue that you can see with your eye. What we’re seeing is all the individual cells and these little tiny red dots are fungus.
Dr. Klein is especially interested in studying how fungus induces asthma in people and how your immune cells respond to a pathogen within your lung. And by understanding that more– By the basic understanding of how these things get arranged and these green guys are actually what are called– These guys, right here. These are immune cells that have infiltrated the lung and become activated in the presence of fungus. And he is trying to understand how the spatial orientation of the fungal infection activates and exasperates an asthmatic attack.
So this is live tissue taken over about 30 minutes in time and we were able to see that the spatial orientation is in fact important for the activation of these immune cells. This isn’t so dynamic though. It’s not like a movie, we can’t watch it in person. We can’t really see the immune cells coming in and out. But if you wanted to start doing that, you can do that rapidly on a new microscope, or a modern microscope.
So this is taken out of Melissa Harrison’s lab. She’s in biomolecular chemistry and she’s extremely interested in how maternal factors drive development. So maternal factors are inherited by the mother. And they are incredibly important in the first steps of development in an embryo. So this is in drosophila. These are CRISPR-engineered organisms and what you’re going to see is– You’re going to see single transcripts gets transcribed, that’s the green. So that’s DNA getting transcribed in a living organism in real time. The red, for those of you guys can see, mark the histones of the DNA. We can push this a little bit farther and we can push this into more of an adult drosophila that’s further along in the development.
And Dr. Huang, Zhen, and he’s in genetics…
Sorry.
…is explicitly interested in studying the organization of how a neuron comes onto a muscle and causes contraction. And is also interested in these sort of, what they’re called neuromuscular junctions where neurotransmitters are released into space. He marks these neurotransmitters by calcium channel, and you can multiplex this over time. And you can watch the synaptic, these junctions be activated and release neurotransmitter in real time. So this is taken from
This movie’s going to be taken from Dr. Kate O’Connor-Giles’s lab. She was in genetics and moved to Brown last summer. And what you’ll see is that– So that’s the same junction, it’s just zoomed in now. And the spikes are actually single synapses being released. She’s incredibly interested in understanding the role of the synaptic heterogeneity, so the probability that a single synapse will release and how that develops neuronal circuits that are important for learning, or important for anxiety-related disorders. So she was able to map each individual active zone with events in real-time, and then look at the probability of release and then correlate this to behavior.
If you want to study this maybe– We had mentioned the word vesicles earlier. I don’t think it got captured by the broadcast. But in my own work, we were studying a vesicular trafficking at the plasma membrane, so the release of hormones into the extracellular space. You can think about it; insulin being released out of a cell. And how that gets regulated by the protein environment around it.
So if you look, if we go to the next slide, each one of these green markers are a vesicle, small packet of hormone, and when they get bright, it means that the vesicle’s opening into extracellular space and the content is being released. You can simultaneously image at the same time another channel. So in this case this is the cytoskeleton of the cell. And you can watch these at the same time and understand the dynamic behavior between one protein and another protein.
But there’s a catch, right?
This is super– The red on here is pretty diffuse looking, right? And that’s because the diffraction limit of light is not allowing us to see these fine structures. So, I think it was in 2014, the Nobel Prize was won by a number, by Stefan Hell, William Moerner, and Eric Betzig for the development of super-resolution microscopy. And that breaks that spatial diffraction limit of light. And so, during that time, we had known about it. This is prior to the work being done, or the Nobel Prize being awarded.
Here at the Optical Core, we had developed a technique based on their technique in order to study the cytoskeleton of these cells in greater detail. And so what you do under this is that you take a movie very, very fast. So this is 100 frames per second and it’s hard to see, but there’s individual blinking events. And what you can do is like– That’s a single molecule blinking in time, and you can use math to essentially plot that molecule in space and fit it with a mathematical function that allows you to estimate where that molecule is in space, with higher resolution than what the diffraction limit gives you. And so if you do that and you add up– It’s essentially pointillism, I like to think of it like that. So, if you do that, over time you can start seeing
This is the same image. And you can start zooming in and you see these really fine structures which are starting to get blurred out because this camera has pixels and is binning in the information. And you can zoom in even more. And what we know is that the individual vesicles lie within these spaces, right here, and that over time, if you trigger the cell to release its hormones, this network, the big fibers get cut and they get dissolved, and then they come back. And that’s very important for the regulation of the amount of neurotransmitter that’s released from each individual vesicle and then total number of vesicles that are released over time.
So, with that, I just sort of want to end with one of my favorite quotes. So, “I have spent “more time than many will believe “making microscopic observations, “but I’ve done them with joy “and I’ve taken no notice to those “who have said why take so much trouble “and what good is it?” That was taken from Antonie van Leeuwenhoek and I still think it applies to me today (chuckles) 350 years later.
And with that, thank you for your attention.
(applause)
Search University Place Episodes
Related Stories from PBS Wisconsin's Blog

Donate to sign up. Activate and sign in to Passport. It's that easy to help PBS Wisconsin serve your community through media that educates, inspires, and entertains.
Make your membership gift today
Only for new users: Activate Passport using your code or email address
Already a member?
Look up my account
Need some help? Go to FAQ or visit PBS Passport Help
Need help accessing PBS Wisconsin anywhere?
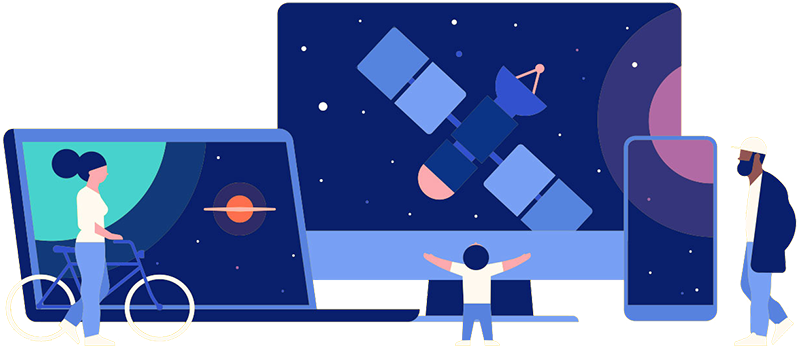
Online Access | Platform & Device Access | Cable or Satellite Access | Over-The-Air Access
Visit Access Guide
Need help accessing PBS Wisconsin anywhere?
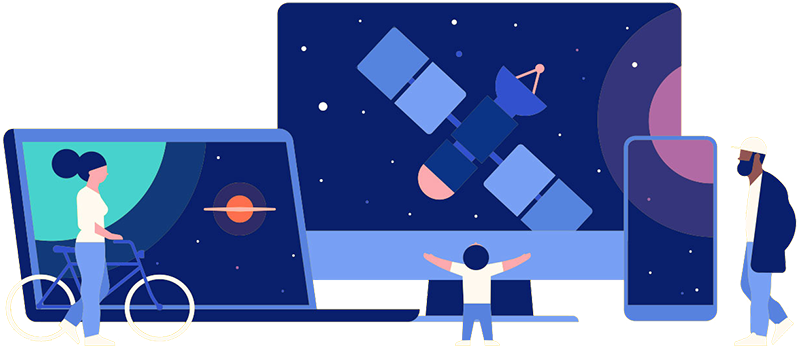
Visit Our
Live TV Access Guide
Online AccessPlatform & Device Access
Cable or Satellite Access
Over-The-Air Access
Visit Access Guide
Follow Us