– Good evening, my name is Jim Lattis, and here at UW Space Place, this is one of our guest lecture nights, and tonight, I’m very pleased to introduce one of our physics professors from UW, and his name is Baha Balantekin. And Baha, when this news first came out, about, maybe it was a year ago or so, that the collision of neutron stars had been discovered by gravitational waves emitted by that collision. I asked him if he would come give a talk about this, this very interesting discovery, and he kindly agreed to do that. So I’d like you to welcome Professor Balantekin.
(audience applauding)
– Thank you Jim, it’s a pleasure to be here. And today, I would like to tell you about, the title is there, “Gravity Waves, Gold, and Neutron Stars,” but I really will tell you about the origin of all the elements, where different elements are made. But first, let me put everything in the right setting. We are living in a quite exciting time. We have really revolutionary advances in astronomy. They are taking place in astrophysics and cosmology. And these advances are motivated by really high precision instruments, like satellites, like the series of satellites from COBE to WMAP and Planck, and of course, very powerful telescopes, which are on the Big Island of Hawaii, like Subaru and Keck and perhaps even better, the 30-meter telescope being built in the future. So this is one component of the revolution that we are in the middle of in astronomy, and astrophysics, and cosmology. A second part of this revolution is guided by the state of the art nuclear and particle physics facilities where you study the microphysics of the phenomena that take place in the universe. And you probably notice, you notice the very worried theorists about how difficult it will be to interpret the data from those experiments. And to this list, we very recently joined another observatory of sorts, the gravitational wave observatory, LIGO, as you can see at two perpendicular arms. And this is what we call today multi-messenger astronomy. That is to say doing astronomy using all possible venues. Well, let me go back about 18 years, beginning of the century, when the U.S. National Academy of Sciences produced a report called Connecting Quarks with the Cosmos. And they listed about 11 science questions for the new century.
And two of those 11 questions are relevant to what I’m going to talk today. One is, “What are the masses of neutrinos, and how have they shaped the evolution of the universe?” And the question 10, “How were the elements from iron to uranium made?” Including gold, and silver, and platinum, and so on. So, the question is, how do you cook the elements around us? Well, of course, you need– When I say elements, I’m not talking about the electrons around the nuclei, I’m actually talking about how you form the nuclei of those elements. Because once you form the nuclei, electrons get attracted and stay around. So, I mean, the nuclear table is there, and you have my favorite French cartoon character, Obelix, actually producing a brew of the elements. Well, how do you make these elements? Well, of course, we start at the beginning.
In the Big Bang, you do produce some elements. You produce, of course, hydrogen, because what happens is, in the very early universe, you have quarks and gluons and so on, and at some point, they put themselves into protons and neutrons, and of course, hydrogen nucleus is a proton and it acquires electrons around, so you actually do make some hydrogen, quite a bit hydrogen. It is actually a bit harder to form neutron in the early universe, as compared to proton. The reason is, neutron is a bit heavier, a little bit heavier. It takes a bit more energy to actually form a neutron from its constituents. So, there are fewer neutrons in the early universe than protons. That means, eventually, you can form helium, the alpha particle, which is two protons and two neutrons, or deuterium, which is one proton and one neutron, in the early universe. But it gets harder and harder as you go beyond that. Well, another reason is the different nuclei have electromagnetic interaction between them. They repel each other electromagnetically.
The heavier they are, the stronger they repel. As a result, it is real hard, it is real hard to form nuclei with a lot of protons or neutrons. So then, this is all you can do in the early universe. Basically, 75% hydrogen, 25% helium, approximately, and a little bit of everything else. So, when you do that, then of course, you form stars from these elements. These are very, very big stars, that what we call in astronomy, Population III stars. These are really big and astronomers call them metal poor, but in the astronomy parlance, metals mean anything that’s not hydrogen or helium. So, like you are breathing metals, and you are all made out of metals. Sounds like Doctor Who, right?
(audience chuckles)
And, so, these stars, of course, evolve, and I’ll tell you about the evolution of the stars and how actually they form some of the elements. And eventually, they go supernova, and they distribute in the universe the elements that they formed. And these elements, they go to supernova. And these elements are no longer just hydrogen and helium, but all the other, all the elements all the way up to iron. Heavier elements between helium and iron, they are produced. And, of course, once these new elements are produced, they again get together because of gravity, they form stars, these stars evolve. These are called Population II stars. They are still metal poor, in the sense that they really do not have still too many heavy elements. And, of course, these stars, some of them become supernova, and they form the elements like uranium, europium, thorium via the so-called r-process, and some of them produce some other elements like barium, et cetera, via the so-called s-process. These processes are called R and S, R stands for rapid, and S stands for slow.
It is just the capture of neutrons on seed nuclei. You keep capturing neutrons and form heavier nuclei. Since neutrons are neutral, they do not worry about this electromagnetic repulsion, so it’s easy to capture them on the nuclei. As a result, you can actually produce them. These are either– if the capture happens rapidly, we call it r-process. If the capture happens slowly, we call it s-process, obviously. Well, s-process takes place over millions of years, because its slow, it takes its time, the stars evolve and so on. But the r-process is very rapid. For r-process to happen, you need violent phenomena in the cosmos. And here is a periodic table, an unusual periodic table, in the sense that it actually tells you where different elements are made, where different elements are made.
And, as you can see, that some of the elements are made in the early universe in the Big Bang, some in the large stars, some in supernova, and so on, and I will talk about that. So, let me go back to early universe a little bit more and tell you what we actually measure. There’s still a problem with our understanding of the early universe that I should mention. Well, what we do, we measure the leftover radiation from the early universe. That’s something we can do with those satellites, WMAP, COBE, and Planck type satellites. But when we measure the leftover radiation from the early universe, we basically are looking at the point of last scattering of that photon, that piece of light, that come to our instruments. Well, because when you look at the sky, when you look at the clouds, you really do not see everything, but you see where the photon that came to your eyes last scattered from the cloud, so you see it white. And that point of last scattering happens not during the Big Bang. Big Bang nucleosynthesis, element formation in the Big Bang happens in the about first three to four minutes after the Big Bang. Whereas the point of last scattering happens about 300,000 years later.
It may sound like a long time, but that’s nothing, of course, for the age of the universe. But nevertheless, that’s what we do. So, there are a number of reactions that can take place between different nuclei in the early universe. It doesn’t really matter what they are, but if you look at the right, it tells you the observed abundances of those nuclei mentioned, helium, and helium-3, and lithium and so on, and deuterium. The various lines give you the abundances. Of course, we do not know how much material there was, what the density was, in the early universe. So, you calculate this as a function of density, along the horizontal axis. However, we can measure the density in the early universe with those satellites. The WMAP, Planck, and COBE measure that. And the vertical line is where they actually measured the density. Well, as you can see, it agrees very nicely. There are places where you get the right amount, except lithium. Lithium is a problem. In particular, this is the problem of the lithium. We think that if you look at the earlier stars, two astronomers called Spite and Spite. It’s pronounced spit not spite, which is probably better.
They observed that as you look at the earlier stars, that is to say, probably what you observe is primordial, that’s to say the stars with a high surface temperature, you see that there’s a lot of lithium-11 and that’s probably primordial. That’s observed in the old halo stars. But what’s observed in the old stars is a lot less, about half or a third of what the Big Bang nucleosynthesis predicts. So, we still do not know, for example, where the lithium is made. This is one of the puzzles about where elements are made. But then, as I said, some of the elements are made in the stars, so let’s talk about evolution of the stars. And this is an old question, because our closest star is the Sun, and of course, people wondered where the energy of the Sun come from. Sun comes and heats us.
In 1854, von Helmholtz argued that the energy’s gravitational. Basically, as the Sun shrinks, the gravitational binding energy is converted into light and heat and comes and heats us, which is nice. However, if you look at the size of the Sun, which we can measure, even back then, that would last about 200,000 years. So, if that were the case, the Sun couldn’t be older than 200,000 years. Well, already, there was fossil evidence that the Earth was older than that, so this was a big paradox. Earth was two million years old. According to that hypothesis, Sun was only 200,000 years old, which didn’t make sense. Well, this actually changed when Einstein came up with his famous formula, E = MC2, that is, to say you can create energy from nuclear fusion. Well, and it turns out, it was Sir Arthur Eddington in 1920s who said that perhaps it’s the nuclear fission that powers the Sun. Well, first of all, why do we need energy for the sun to be powered? Well, if you think about this, if you drop objects on Earth, they fall. You know, because everything, there’s a gravitational attraction of the center of the Earth, and it pulls everything towards itself. Well, so, you may ask, why doesn’t an object as big as the Sun doesn’t fall onto itself? It should. Well, something must be preventing that fall, and that is the nuclear energy generation at the center of the star, that compensates gravity. Well, Arthur Eddington, of course, was not easily believed.
And people said, “Well, Sun is not really too hot for this to happen.” So in Victorian, nice Victorian English, he said, “We do not argue with the critic who urges that the Sun is not hot enough. We just tell him to go and find a hotter place.” In modern English, we would say, “Go to hell,” of course. Well, but then, eventually, it was Hans Bethe, the gentleman you’re seeing on the left here, figured out the processes that actually form the source of the solar radiation, and, of course, he got the Nobel Prize for it. There’s an actually interesting story. When he was dating his wife, Ruth, they went out to look at the stars, and his future wife told him, “Well, look, aren’t the stars beautiful? They’re shining so well.” And Hans Bethe says, “Yes, but only I know how they shine.”
(audience laughing)
You know, it just– And so, this picture in the middle is also Hans Bethe. It’s hanging in the Max Planck Institute in Germany, basically telling him how he made the Sun. Well, the interesting thing is, the nuclear elements, nuclear fusion, that powers the Sun also produces neutrinos, as you can see, those little particles that interact very little. And those neutrinos come from the center of the star. They come from the center of the star, where nothing else can reach. None of our other probes can reach, so they’re a perfect way of looking at the center of the star. Neutrinos are weakly interacting particles. Their interactions are very feeble with everything else. They do not have electric charge, so they do not feel anything else around.
They just zip from the center to the surface in about two minutes. And then, they come to us in about eight minutes. In about two seconds, and then they come to us in about eight minutes. So, when you look at the neutrinos coming from the Sun or any other star in the future, you really looking at the core of the star, in real time, more or less. Whereas light, light is like a politician in a fundraiser. It likes to interact with charged particles, electrons and protons. So, it stops and shakes hands with every charged particle it encounters. So, it takes a long time for the light to leave where it was produced to come to the surface. It actually takes about 200,000 years. So, the solar light we see now is 200,000 years old. So, for all you know, the Sun may have been dead, if you don’t see the neutrinos. But if you see the neutrinos, then the Sun is working.
And the first measurement of solar neutrinos took place by Ray Davis, the gentleman on the right of the slide, and John Bahcall, the theorist. In 1964, they wrote a paper to see into the interior of a star and thus verify directly the nuclear energy generation. Well, Ray Davis actually did the experiment in this location. This is an old gold mine. This is the first mention of the gold in this talk, Homestake in South Dakota. And you see the mine shaft there, and we’ll come back to that mine shaft later. It still is operating as a physics, or astronomy place. So, he went down, all the way down. He needed a location where he can go way down, but because the surface of the Earth is a very dirty place, it is full of radiation, don’t tell anyone. So, any signal that you try to get from the stars, like the neutrinos, disappear. You cannot see it. You have to go way below, inside the Earth, so that the Earth above you will filter all the junk you do not need and you will also see the signal that wouldn’t care about the junk, and that’s the neutrinos. So, he went to this mine, and he did a very nice measurement. He eventually got the Nobel Prize, of course, for it. So, he got gold. The Nobel medal is made out of gold.
(audience chuckling)
But, you know, the detector he used was dry cleaning liquid. It was cheap to find, carbon tetrachloride, that’s what dry cleaners used to use, not anymore. It produces cancer. So, it was cheap to get and so on. But, of course, his experiment was difficult to make, I mean, in this mine shaft. Better experiments were done afterwards, and they found a cheaper material. What’s the cheapest material you can find? Water. So, they built a detector under the Japanese Alps in western Japan, based on water, and they were able to detect neutrinos coming from the Sun. And this is the detector that you see. It’s as big as a football field actually. And you see the little rubber dinghy with three people on it? They’re going around fixing the phototubes that detect the photons. Basically, what happens is that when the neutrino comes and hits an electron in the water, it makes electron move faster than light does in water. When a charged particle moves faster in a medium than light would move in that medium, it emits the Cherenkov radiation in a cone, as shown there, and then you actually see the radiation, or the light, coming from that motion, the Cherenkov light. It’s the same light that you see in the pool of a nuclear reactor, that blue haze is the Cherenkov light.
And there, the plot shows the Cherenkov light coming from the Sun from the solar neutrinos as a function of the angle. As you see, as you look at towards the Sun, there’s a beautiful peak, the Sun is working. So, they got that. And this is the picture of the Sun using neutrinos instead of photons, perhaps not very commonly seen. Okay, so, the elements up to iron are actually made in the stars. It’s easy to make them because every time you form an element heavier than hydrogen, all the way to iron, you release energy. Remember, you need to release energy to compensate gravity, otherwise, it wouldn’t work. But once you’ve reached to iron peak, these are the binding energies of various nuclei, once you reach to iron peak, you can no longer actually produce energy by nuclear fusion. You actually need to put energy to make things fission, for example. It’s actually not iron, it turns out. I call it iron peak, not exactly iron. It’s nickel and cobalt, which actually is observed. They are both observed in all supernova. So, you need another process, and that is the R- and s-process to produce those. And this actually was well formulated by these four people, Margaret Burbidge, Geoff Burbidge, Bill Fowler, and Fred Hoyle.
And you see them in front of some instrument, I don’t quite know what that instrument is, but this their paper, and you can see they like to quote Shakespeare about stars. This is actual paper, this is actual quote. And basically, what astronomers do, they look at these elements formed either in the early universe or in the stars and try to get what they were made of. And, by the way, I actually have some affiliation with the National Observatory of Japan near Tokyo, and this their publicity slide. They produced a slide like this where elements are made. As you can see, only lithium is not known. This is not quite right. This is, perhaps, a bit too enthusiastic. Okay, so let me go back about the stars. How do stars work?
Well, stars actually– This diagram is basically the life cycle of a star. The stars start as 75% hydrogen and 25% helium, and they convert the hydrogen into helium, produce energy and compensate gravity. But at some point, they run out of hydrogen fuel. When that happens, when that happens, you have all helium, the star actually collapses a little bit and then expands. It collapses so that its central core temperature goes up, so it can start igniting helium, and that’s what we call red giant. Eventually, you keep igniting heavier and heavier elements, like you start burning carbon and then you start burning magnesium, because you form magnesium from two carbon fusing, and so on. And every time you produce heavier elements, it goes faster and faster. The production, the conversion of hydrogen into helium will take millions of years. But the very last stages take days. And here is an example of what happens towards the end.
Basically, in the center of the star, you are producing heavier and heavier elements. At some point, you reach the iron in the core of the star, and the outside, other parts of the star, you’re still producing a bit lighter elements, but at the center, you form iron. When that happens, you can no longer fuse iron nuclei to produce energy and compensate gravity. When that happens, the star collapses, the core collapses. This is called a core-collapse supernova by physicists. Astronomers call it supernova of Type IIa. I think physics nomenclature is a little bit more colorful. It’s called the core-collapse supernova, and, of course, what happens is that during the collapse, the electrons in the material get captured on the protons, and when that happens, you form a neutron, and you emit the excess energy as a neutrino. Well, it turns out, you produce a lot of neutrinos in this process. As the star collapses and everything becomes neutrons, you produce a lot of neutrinos.
You basically emit the entire gravitation binding energy of the pre-supernova star as neutrinos. So, core-collapse supernova are like neutrino factories. Now, people are trying to build experiments looking at all these neutrinos from all past supernova. Well, and then, at the center, of course, you have this hot proto-neutron star that you form. Well, here are some nice pictures. This is a recent supernova remnant seen by the Hubble Space Telescope. And you can actually look at the supernova in various wavelengths, and this is the famous supernova observed by Chinese in 185 years before Christ and in X-ray. This is the remnant of that supernova. And this is Kepler’s Supernova. Kepler has discovered, or observed, the supernova in 1604. This is the X-ray remnant of that supernova. And this is the estimated intensity of the brightest supernova ever, which took place in 1066 AD, and from the location of National Observatory of Turkey in Antalya. Now, National Observatory of Turkey did not exist in 1066.
(audience laughing)
So, this picture was made by an astronomy student. He put the supernova where it should be. That’s Mediterranean Sea, because that observatory is on a high mountain looking at the ocean. And then he calculated where the stars would be at that time, from their current locations. So, he shifted the stars back to where they would be, and that’s the image that he created. It’s interesting. So, when the supernova collapses, and at the center, all the neutrons come together to form what I would call a proto-neutron star, a hot neutron star which eventually will cool to be a neutron star. But of course, you cannot keep pushing material down and down. At some point, there’s a bounce. Well, you know if you–
The bounce creates a shockwave. The shockwave goes out and hits the remnants in the other part of the supernova, basically nickel and cobalt, ignites them, excites those nuclei, and produces light. And this is that light that we see as a supernova light. It’s a rather small one. Here’s an example of the supernova shockwave, but of course, at the center of the star, this old, lonely proto-neutron star sitting, which eventually will cool. Okay, here is another way of looking at where the elements are made in the Big Bang, in the older stars, and r-process, and what we observe in the solar system.
This r-process, or the rapid neutron-capture process, may happen in a number of locations. For example, it may happen in a supernova. Because in supernova, especially near the proto-neutron star, you have a lot more neutrons than protons, so it’s easier to capture neutrons on the protons. There are a lot of them. And seed nuclei. Or in the merger of neutron stars. They are both possible. And, as a matter of fact, today, we believe some elements are formed in the supernova and some elements are formed in the merger of neutron stars. But here is the elements observed in a number of different stars. These are the phone numbers of these stars that astronomers gave, or zip codes, if you wish.
I’m not sure the mail will get there yet, but– So, these are the observations of the r-process elements, the relative abundance as a function of the atomic number of the elements they observe. And they’re scaled. They’re all scaled to the solar abundances. As you can see, there is a tremendous universality. They all look the same. They all follow the solar abundance. Of course, you have to scale, because amount of material you get depends on how much material there was in the star, how big was the star, how small was the star, and so on. So you have to scale, so, what really matters is the relative abundance of one element versus other, not overall abundance. So, if you scale, you see perfect agreement with the solar system abundances of these elements, which suggests a rather universal mechanism that produces them. Now, so, everything I said is fine, but you have to do observations.
We actually have seen the light from the supernova for many times. And very recently, that is not that recent, in 1987, we were able to see neutrinos coming from a supernova, collapsing supernova. You can see there. The Japanese writing says background. That’s what you get if there’s nothing happening. And suddenly, you have an increase in the number of events, and that is the supernova, neutrinos. As I said, these are the energetics of the supernova, and 99% of this energy’s carried away by neutrinos and antineutrinos. So, this is a beautiful watercolor I really like from the science magazine. So, Earth is being bombarded by neutrinos, not only from our Sun and distant stars, but also from the supernova. But if you really want to– We try to observe supernova with neutrinos, that’ll tell us how the elements are formed inside a supernova. But if you really want to observe a supernova with neutrinos, you’d better know what neutrinos do inside the supernova. I like this picture. I like baseball. (audience chuckles)
As I told you, remember that mine shaft. Well, that mine shaft in South Dakota, and you can see the figure there on the right-hand side. It’s still there. And you go down, and there’s a laboratory there still. And they are planning to send the beam of neutrinos from Fermilab to that facility, to study the properties of these neutrinos. Basically, I don’t know if you had been to Fermilab, but this is the main building in the Fermilab. It’s not too far away from here, depending on how fast you drive, it’s two, two and a half hours. So, they will produce a beam of neutrinos. And the beam of neutrinos will first be produced and, of course, you somehow need to store them in a big ring, and then, even a bigger ring. And those are the protons that you are accelerating. And then you have these protons hit some target to produce the neutrinos. And there you go; the greens are neutrinos. And there’s a Near Detector that measures how many neutrinos you’re sending. And the neutrinos will travel through the Earth about 700 miles, and all the way to that lonely mine shaft. And they will be detected there. And, of course, perhaps a supernova will go off at the same time, and the neutrinos from the supernova will come and be detected. That’s how the whole thing works. And these detectors are big. You see the size a person, but the detectors are much bigger than the people.
And eventually, you will get a good understanding of these properties. And you get the neutrinos. So, let me now switch gears and talk a little bit about this gravity wave and see what we observe there, okay? So, this is another way to look at the universe. It basically has these two arms that go mile each way, a little bit more than a mile each way, and they are perpendicular to one another. Why do we have this? Well, this slide actually nicely explains how they work. When two, say, black holes, before they observe the merger of neutron stars, they observe the merger of black holes. Let’s say you have two black holes, these are small black holes, not big ones. The big ones at the center of our galaxies and other galaxy, in our galaxy and other galaxies, but these are small black holes. So they start spinning around each other, they eventually merge. And during the spinning process, they disturb the spacetime and produce gravitational waves. And basically what happens, there are these two perpendicular arms, the gravitational wave eventually reach us, the Earth, and it hits those two different arms. Basically, what happens though, they change the length of the arms in slightly different ways, very, very small difference. And they measure this change in the length by sending photon beams, and having them reflected, and looking at their interference.
This is actually a very, very impressive experiment, I must say. And, of course, this had been observed. What they have is, they have two of these detectors in the United States. One is in Hanford, Washington, the old nuclear waste site. Don’t tell anyone. And the other one is Livingston, Louisiana, near New Orleans. And what they had seen in these two detectors separately as a function of time, when the gravitational wave from the neutron star merger hit them. Well, of course, you have to be careful. You can’t have a single detector because, who knows, you have these two large arms, but a big heavy truck moving around may shake them, and you may mistake that shake as the gravitational wave. So, what you need is two very distant locations, where there is no correlation between the trucks driving by, and so, you want to be able to observe the same event in two different locations, and that’s what they did. However, you have to be careful. The gravitational waves do not travel with infinite speed.
They also travel with the speed of light, because nothing can travel faster than light in vacuum. So, they don’t reach those two detectors at the same time. There’s a slight time difference. So, you have to shift them and put them on top of each other, and you get exactly the same signal. So, these detectors, these two arms, can actually nicely measure the gravitational waves coming from black hole mergers. That was about a year and a half ago, and as you know, they got the Nobel Prize for it. But more recently, about a year ago, this is almost two years ago, more about a year ago, they were able to observe the merger of two neutron stars. And this is basically how the process looks like. So, the two neutron stars are orbiting one another, and on the left, you see how the matter is distributed. On the right, you are seeing the gravitational waves that are being formed and emitted. As the stars get closer and closer, matter starts getting transferred from one to another. You basically form one large object, and there’s an incredible amount of gravitational wave emitted as a result. So, that is… basically what they had seen. This was in August 17, 2017. It was seen in the United States in those two detectors, and a similar detector in Europe, in Italy, Virgo detector. And so, they were actually able to observe the merger of two neutron stars. Now, people many times tell me, are you excited that Einstein’s theory of gravity, general relativity, was confirmed. I must say no. Because general relativity was confirmed in 1917 already.
There are many, many places where we know general relativity works extremely well. So, it was nice that we were able to observe, we were able to believe that gravitational waves are formed, but what’s really tremendously exciting about this experiment is that they can measure a change in the length of very, very small nature. That was an amazing experimental feat. It’s unparalleled in the history of experimentation. They were able to measure changes of a fraction of a micron, even less. So, that was the amazing part. Well, the interesting thing, though, about neutron star mergers is that not only they were able to see the gravitational waves from that merger, but also saw the light. There was an electromagnetic counterpart that was observed in a lot of observatories around the world, the Fermi Gamma-ray Space Telescope and so on. They actually saw an electromagnetic counterpart. They saw photons together with the gravitational wave, more or less simultaneously. This is, of course, heralded as the beginning or the triumph of the multi-messenger astronomy. And if you look at that light that was observed, this is the intensity as a function of time, days since merger. And the colors there you see is the true colors, how the color of the electromagnetic spectrum that you see change as time went on. It quickly became quite red.
Well, where is this coming from? It turns out, we are producing elements in these neutron star mergers. We are producing nuclei, heavy nuclei, with the r-process. We keep capturing neutrons because neutron star mergers have a lot of neutrons, no question. You keep producing r-process nuclei, but these nuclei are quantum objects. They have energy levels, and they are produced in excited states, and they decay into ground state, and you can actually see the photon coming from those decays.
But you have to be sure about this. So, what you do, of course, for example, this is the light curve coming from this merger event. And the data points are given there. There are several lines there, but the blue line is the simulations of element formation, r-process formation. And, of course, as I said, there could be some nickel and other elements formed from the earlier supernova, which means that they will still be hanging around the neutron stars a little bit, and they may be excited too, and you may confuse them. So then, the other lines, the dashed lines are heating of nickel and cobalt. And you can see the data really agrees well with the r-process element formation. Also, you want to know how much element was formed, so they also make simulations. The data, look at only the top graph. The data is again shown there as squares and circles, and you can actually fit it with the amount of heavy nuclei that were formed.
The blue is 0.01 times the mass of the Sun amount of heavy nuclei. And the orange one or the brown one, is about 5% of the element formation. So, the data actually tells you, not only you are forming these heavy elements, but also forming how much of these elements, maybe about 5% of the solar mass. So then, you can go ahead and so simulations, see what you get. Now, all this data tells you that the heavy elements are being formed. It still doesn’t tell you what is being formed. You have to look at that separately. You have to figure out what’s being formed separately. Now, in astronomy, many times you can tell which elements are out there by looking at the atomic spectroscopic lines. So, you can do spectroscopy.
You can look at the atomic lines, and then compare them with your catalog of atomic lines and tell which elements were there, which elements you are looking at. But this star was so far, so all the light coming from it was Doppler broadened. This is the Doppler effect that you know. Ambulance comes to you and goes away, you hear very different frequencies. It broadens. So, all these lines just mix with each other, so it’s very difficult when the event is this far, very difficult to actually look at individual lines. If it’s closer, maybe they could, but very difficult to look at. However, you can also look at, not just the magnitude, but the color of the optical emission. And that’s actually sensitive to the composition of the ejecta, it’s sensitive to which elements you’re forming. And here is a sample calculation.
This is the so-called lanthanides. I’ll show you what they are in a second, but this is a bunch of elements that are in the periodic table, and it tells you what fraction they are being produced and the color of the actual light curve that you see. And on the right, you see what you see optically, and that is to say, with your eye, and what you see in the infrared. So, it turns out, if you form these lanthanides, the light is shifted towards infrared, it reddens, the ejecta reddens, and you have a significant amount of infrared light coming in, which is exactly what the observatories saw: significant amount of reddening towards infrared. And that tells us that the elements you formed in this neutron star merger are lanthanides, the elements that are circled there, lanthium and then all the way, all the others. I mean, you know some of them, perhaps, samarium, europium, and so on. So, we have signature of these of these elements, direct signature of formation of these elements in this neutron star merger. Unfortunately, no signature for gold. Gold doesn’t give you the reddening, and we see a lot of reddening. Maybe some gold was formed, but it was masked by the huge amount of lanthanides that were formed.
So, I think it is safe to say, both neutron star mergers and core-collapse supernova do produce those heavy elements. Just what is produced where is the question, and that’s, I think, what we need to understand in the decades to come. So, I think I should stop here. Thank you very much.
(audience applauding)
Search University Place Episodes
Related Stories from PBS Wisconsin's Blog

Donate to sign up. Activate and sign in to Passport. It's that easy to help PBS Wisconsin serve your community through media that educates, inspires, and entertains.
Make your membership gift today
Only for new users: Activate Passport using your code or email address
Already a member?
Look up my account
Need some help? Go to FAQ or visit PBS Passport Help
Need help accessing PBS Wisconsin anywhere?
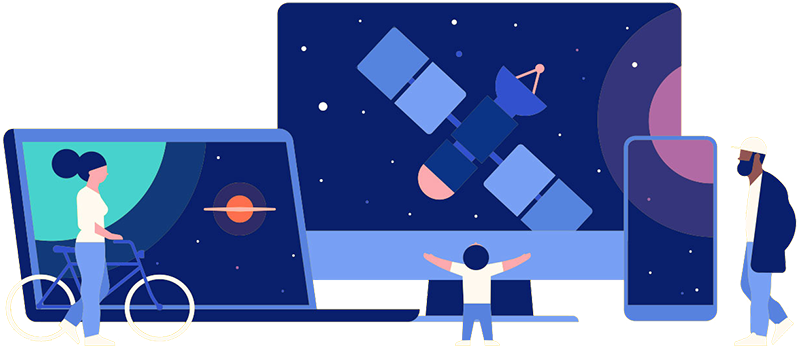
Online Access | Platform & Device Access | Cable or Satellite Access | Over-The-Air Access
Visit Access Guide
Need help accessing PBS Wisconsin anywhere?
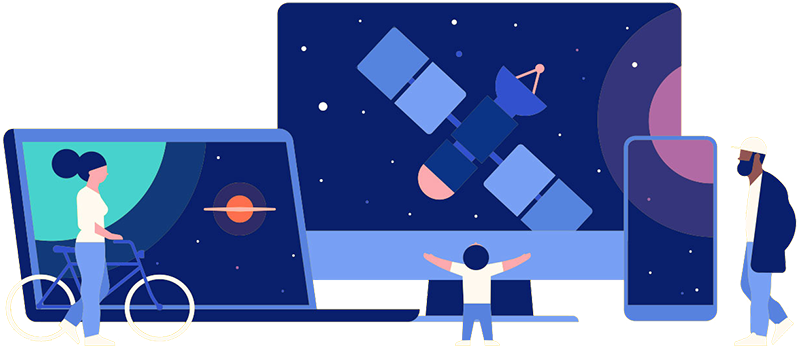
Visit Our
Live TV Access Guide
Online AccessPlatform & Device Access
Cable or Satellite Access
Over-The-Air Access
Visit Access Guide
Follow Us