[Tom Zinnen, Outreach Specialist, Biotechnology Center, University of Wisconsin-Madison]
Welcome everyone to Wednesday Nite @ the Lab. I’m Tom Zinnen. I work here at the U.W.-Madison Biotechnology Center. I also work for U.W.-Extension Cooperative Extension, and on behalf of those folks and our other co-organizers, Wisconsin Public Television, the Wisconsin Alumni Association, and the U.W.-Madison Science Alliance, thanks again for coming to Wednesday Nite @ the Lab. We do this every Wednesday night, 50 times a year.
Tonight, it’s my pleasure to introduce to you Shanan Peters. He’s a professor here in the Department of Geosciences. He was born in Lancaster, Ohio, and went to –
[audience member interrupts]
– went to high – would you like to introduce? [laughs]
[laughter]
And went to school at Fairfield Union High School there, did his undergraduate work at Davidson – Denison University in geology and biology, got his PhD at the University of Chicago in the Department of Geophysical Sciences there, and spent three years at the University of Michigan, and then, 10 years ago, came here to the University of Wisconsin-Madison.
Tonight, he gets to talk to us something that’s really important for those of us who like pizza, and that’s the evolution of the crust. Except for this one is the upper crust of the Earth. Biogeochemistry, it’s not every day we get to have all three words together. It’s impressive that you have an undergraduate degree in both geology and biology.
I remember when biogeochemistry came out as a word. It was like, wow, this is amazing. Rocks, life, and reactions. If only I were -if only I were younger. So, I’m looking forward to hearing about this. Please join me in welcoming Professor Peters to Wednesday Nite @ the Lab.
[applause]
[Shanan Peter, Professor, Department of Geoscience, University of Wisconsin-Madison]
Thank you very much and thank you for braving the elements out there today and – and finding the tornado shelter. Hopefully we don’t have to use it, but it sounds like we’re safe here this evening.
So, I’m going to be talking to you about the long-term view of Earth’s biogeochemical evolution. And, you know, the Earth’s a pretty remarkable place. It’s home.
[slide featuring a photo of the Earth from space]
We’re quite familiar with it, but it’s really weird. And you can see a lot of what makes it weird and distinct deep within our solar system in this image. You know, we have water. That’s not weird. Water is everywhere, but we have it in all three states. And you can see that here. That’s pretty special.
Earth is very bimodal in elevation. We have very high-standing continental crust and very low-standing oceanic crust. That’s weird. We are profound – profoundly bi – bimodal in the elevation. That’s due to tectonics. We have active plate tectonics, and that’s a weird thing. And, of course, we have life, which is maybe not weird but certainly important part of the story we’re going to hear about today. And so, all these things come together in the story I’m going to tell you, and I think the best way to get a handle on why you have to understand all three of these things operating together as one evolving system –
[Shanan Peters]
– why that’s so important.
By asking one simple question. Well, not really simple, but a pretty straightforward question. Why is there so much molecular oxygen in Earth’s atmosphere?
[slide with the photo of Earth from outer space animates on the question – Why is there so much molecular oxygen in the Earths atmosphere? And shows the statistics of 78.1% Nitrogen, a bolded 20.9 percent Oxygen, .9% Argon and .04% Carbon dioxide]
That’s one of the things that makes Earth just weird. And this a rough composition. The Earth’s atmosphere, dry atmosphere. It’s about 78% nitrogen gas, about 21% oxygen, molecular oxygen, O2. Argon and then CO2, which we spend a lot of time talking about for good reasons, is actually a trace gas. It’s not very abundant in Earth’s atmosphere, and we’ve clicked it up a little bit incrementally here. We’re above .04 permanently now, probably thanks to our activity.
Now, just to give you a sense of how weird this is, you need to compare the present composition of the atmosphere to where the atmospheres of planets come from. And that is de-devolatilization of the magmas coming out of volcanoes. In this case, on Earth –
[slide animates on the chemical statistics of volcanic gasses – about 60% water, about 40% Carbon dioxide and trace percentages of sulfur, nitrogen, argon and helium as well as an inset photo appears of a volcano erupting as seen from space]
– they’re coming out of the vents we can see active. And if you look at the composition of gases coming out of the volcanoes looks nothing like our atmosphere. Okay? It’s mostly water and CO2. It turns out this is really variable from place to place from details of geology underneath volcanoes and so forth, but that’s a pretty reasonable composition for average volcanic gas. Mostly water and CO2 and a few other trace gases mixed in there.
Okay, so our present atmosphere looks nothing like the source of gases to the Earth’s surface environment. At all. And why is – why is there so much oxygen atmosphere?
[new slide featuring an artists rendition of a prehistoric forest on the left along with the word – photosynthesis! at the top and two photos of Rubisco from green alga at the top of the slide]
I think a lot of people would say, well, because life. And we have this amazing biochemistry tied up in enzyme catalyzed reactions, ultimately sourced from the central dogma playing out to make proteins that do amazing things. And one of these proteins is Rubisco here. And this one’s from green alga, but that’s in the chloroplasts of plants as well.
[formula animates on the slide – 6 parts water plus 6 parts carbon dioxide + energy]
And what is photosynthesis in this Rubisco catalyzed reaction do? Well, it takes the two most abundant gases in volcanic emissions, CO2 and water, mixes it with sunlight, and catalyzes this reaction to drive it –
[an arrow points away from the original formula to come up with the result of the equation which is one-part C6H12O6 plus 6 parts oxygen]
– towards making sugars and releasing as a byproduct molecular oxygen. Okay.
So, it’s certainly the case that photosynthesis –
[the statement Photosynthesis is a necessary condition for generating an oxygen-rich atmosphere animates on the slide]
– the evolution of this key metabolic pathway that takes the most common gases coming out of planets, carbon dioxide and water, harnesses the most abundant energy source in the universe, probably, light from stars, and makes – and makes energy –
[Shanan Peters]
– makes sugars, and releases as a waste product molecular oxygen. Okay, so that’s a necessary condition for explaining why we have so much oxygen in Earth’s atmosphere.
But here’s the problem – I can take this reaction and I can write it both directions. And here we are, we probably had dinner today, most of us, or maybe a beer, and we’re in the process of doing this in reverse. We’re taking and we’re breathing in the product of photosynthesis oxygen, we’re ox – using that, there’s enzymes that catalyze this reaction. You don’t need to have them present. It can proceed inorganically too, if you get it warm enough, that reaction is spontaneous. And it’s –
[slide animates on the chemical reactions over the photo of the prehistoric forest as well as replaces the photosynthesis statement with a photograph of aerobic respiration and a diagram of the Krebs cycle]
– its generating energy and releasing, as a gas, CO2, and we’re exhaling it into the room now. Okay, so this reaction we can write in both directions. You can produce oxygen, but biosphere can also consume oxygen. And that’s what we do back and forth with plants.
Now, to illustrate how incredibly important photosynthesis is but not really enough –
[Shanan Peters]
– we can do this little thought experiment here. Okay? We’re in a nice, little tornado shelter as it turns out. We can seal the doors and we can force this reaction in this direction up on the screen here.
[slide featuring the illustration of a prehistoric forest with the chemical reaction superimposed over it and a new question – What would happen if we combusted every living cell on Earth? and the oxygen forming equation showing the C6H12O6 + 6 parts oxygen going back to 6 parts water plus 6 parts carbon dioxide plus energy]
We can force this reaction, but – which is what we’re doing right now by breathing and digesting and processing our food and drive it this way. But let’s do it for everything except us. Every cell on the planet, every living thing on Earth, all the plants, all the animals, all the biomass on Earth, let’s combust it now, seal the room, and ask the question: What happens?
[new slide with a photo of the Earth taken from space asking the question posed above and the statistics of 78.1% nitrogen, 20.9% oxygen, .9% argon and .04% carbon dioxide]
What happens to Earth’s atmosphere? Would you be scared to unseal the room and go out into this new world? Well, let’s do it. It turns out we have a reasonable estimate of the mass of life, living mass on – on Earth. And this is what happens.
[statistics on the slide change to 78.1% nitrogen, now 20.4% oxygen, .9% argon and now .09% carbon dioxide]
Virtually nothing. You – you would go – you wouldn’t even notice. Now, it would be less green, and you might have trouble finding food unless you were able to synthesize it cleverly somehow, but you would live the rest of your life and not – not be worried about oxygen. Okay, so photosynthesis is not why you have oxygen to breathe. It’s the first step.
[return to the slide with the illustration of a prehistoric forest with the chemical reactions superimposed along with the photos of Rubisco from green alga and the water plus carbon dioxide plus energy equation, but now with the quote, Photosynthesis is a necessary but NOT SUFFICIENT, condition for generating an oxygen-rich atmosphere. (Emphasis added)]
Okay, photosynthesis is necessary, but it is not sufficient –
[Shanan Peters]
– to have an atmosphere like you’re used to enjoying and breathing and consuming as a high energy animal. Okay?
So, what do we need to do to build up oxygen in significant quantities in Earth’s atmosphere or any atmosphere? All we have to do is we have to take this reaction –
[slide featuring the prehistoric forest with the chemical reaction superimposed along with the equation of 6 parts water plus 6 parts carbon dioxide plus energy equaling c6H12O6 plus 6 parts oxygen with a smaller photo of a volcano erupting underneath]
– we’re going to assume that some sort of photosynthesis to generate as a waste product oxygen’s required. Maybe you don’t need Rubisco, but you need some biologically mediated process to do that. It doesn’t happen spontaneously by any mechanism where in significant quantities that we’re aware of. Okay? So, life is present. What we need to do to generate significant quantities of oxygen is make this reaction go this way, turn the Earth green, but not let it go back the other direction. To cut this process off so that it doesn’t get oxidized and consumed by some animal like you and me and we leave this behind. And where does this end up going?
[slide animates on two photos of sedimentary organic matter underneath the C6H12O6 plus 6 parts oxygen part of the equation]
It goes into sedimentary rocks. And I have a few examples here. Afterwards, if you want to come up here and look at them, you can.
[Shanan Peters holding up two sedimentary rocks]
This is why you can breathe the atmosphere. It’s life plus rocks. And it’s not just any rocks, rocks that contain organic carbon. And this one –
[striking the two rocks together making a clinking sound]
– it sounds like a rock, it is a rock, but it’s black. And that black comes from organic carbon compounds that are tied up in here. And this one is important. As – as was mentioned, I’m from Ohio, and unlike Wisconsin, Ohio is at the edge of a sedimentary basin that has a lot of this rock in it. And this particular one – this one is called the Chattanooga Shale. It’s a little south of Ohio, but there’s similar rocks in Ohio of – of the same age, like the Marcellus Shale you’ve no doubt heard about. And this is a fracking target. Okay? This rock [clinking rocks together] has organic carbon that was produced by photosynthesis and locked in this rock, and we go down and get it. So, fossil fuels are hyper-concentrated versions of this organic carbon produced by photosynthesis that’s been locked in the rock record, and we’re getting better and better at finding the nooks and crannies that have lower concentrations of this stuff for better or worse. That’s what we’re doing. Okay?
So, in order to produce an atmosphere like we’re used to, yes, you have to have photosynthesis but that really doesn’t do much by itself. At all. It needs to be coupled to an ability to sequester that organic carbon into the rock record, and we need to do this in sediments through a geological mechanism.
Now, if – if you stop for just one second and think about this, you should have been suspicious of – of the notion that we owe our oxygen to the rainforest or something. It’s not true at all. And – and if you think about it, it – it better not be true because you – a lot of us drove here today, and we’re going to go back outside and turn on our cars and we’re not going to have to look in the backseat and say, like, I’m sorry, Johnny, you’re going to have to hold your breath. You know, we’re not worried about consuming oxygen on industrial scale out of atmosphere, but that’s exactly what we’re doing. There’s so much organic carbon tied up in rocks that it has left behind a huge reservoir of oxygen in the surface environment, and we can see us consuming oxygen and replacing it with CO2.
[slide featuring two graphs from Mauna Loa, Hawaii one showing amounts of oxygen going down and the other showing levels of carbon dioxide going up with the statement – We are burning coal/oil/gas and turning oxygen into carbon dioxide]
This is one of the most compelling lines of evidence, I think, for why humans are the reason for the CO2 increase that you can possibly imagine. Its – you don’t hear it much, but this is it. This is from Mauna Loa. These are actual measurements. This isn’t like a model or something. This is – these are measurements of the gas and little samples taken monthly at Mauna Loa going back actually way longer than this record. It’s – its a rare – you should check it out. It’s an amazing record.
And this is oxygen up here, and this is CO2. And CO2 is going up. Now, this is what we talk about a lot. This has a lot of consequences for the climate. We’re not going to talk about CO2 much today, but it’s going up and – and O2 is going down exactly balancing that because the going up part is us consuming oxygen and burning fossil fuels. Okay?
Now, a lot of – Ive – when I’ve shown this before, a lot of people naturally wonder what this sawtooth is, and I’ll just tell you that this is a signature of the geological arrangement of continents on Earth. We are asymmetric as a planet. And even something like the distribution of land mass has a profound effect on the chemistry of the atmosphere on a seasonal basis as the biosphere blooms and breathes in oxygen – breathes in CO2 and pumps out oxygen in the summer, like it’s doing right now, and then does the opposite in – in the winter. Alright?
[new slide featuring a photo of the Earth from space and the aforementioned question of Why is there so much molecular oxygen in Earths atmosphere? with the elemental statistics of 78.1% nitrogen, 20.9% oxygen, .9% argon and .04% carbon dioxide and now the answer to the question Because so much photosynthetic organic carbon has been buried in the sediments!]
Okay. So, the answer to the question, why is there so much molecular oxygen in the Earth’s atmosphere is, yes, photosynthesis has to happen, but it’s because there’s so much organic carbon that has been buried and sequestered away from the surface environment and put into sedimentary rocks.
[new slide with a white background and a photo of the Earth taken from space and the same elemental statistics of 78.1% nitrogen, a now bolded 20.9% oxygen, .9% argon and .04% carbon dioxide, but now with a new question – WHEN did Earth get its molecular oxygen-rich atmosphere? and a small graph of geological time at the bottom of the slide]
Okay, that’s great. The next question, then, is when? When did we get this crazy atmosphere that has this incredible abundance of molecular oxygen that was pumped into it because we buried organic carbon and basically made fossil fuels? Okay, so when did we get it?
[new slide with a the same small geological time line at the bottom, but now above it is a tree diagram of the origins of different genomes, orders, and classes of different bacteria]
I know this is kind of hard to see just based on the colors here, but I’m going to be showing this timescale a lot. And just to get you oriented, these are billions of years ago here –
[using mouse pointer to point at the geological timeline at the bottom of the slide]
– and these names are – are time intervals that are, sort of, the major subdivisions. And Phanerozoic [far right of timeline] just means visible life. Okay? So, this is the time when animals are around. Protozoic [middle of the timeline] means – means, sort of, first life. We know it’s actually not the first life, but that’s the historical name that this time interval has. This is the first life. And this is – there’s no – theres very few animals until the very end of this here. And then this says Archean [far left of the timeline], which is the earliest part of Earth’s history here. And, actually, it goes back to four-and-a-half billion years, but we’re going to unceremoniously truncate that part of the hot, early Earth.
Okay. So, the first part of when did Earth get its molecular oxygen? Well, life has to be part of the story, right? No oxygen in significant quantities, molecular oxygen in significant quantities until we have this metabolic pathway in place. And there’s been a lot of work on this. This is a – a very recent paper in Science that looked at the genome that these – that these cyanobacteria carry around with them. And that – and the molecular distance between the key enzymes that drive the basics of the cell record distances that tell us something about relationship, and that relationship is shown here.
[using mouse pointer to circle the tree diagram]
And what these authors have tried to do is – is identify when oxygenic photosynthesis within this clade evolved. And that requires calibrating these distances with constraints from the fossil record for time. And when you do that and lay that on this, this is what it looks like approximately. Cyanobacteria and oxygenic photosynthesis with that lineage specifically is very old. Okay, this molecular estimate suggests by 2.3 billion years, 2.5 billion years, or maybe even earlier there were cyanobacteria on Earth making molecular oxygen by photosynthesis with the same enzyme that they’re doing today. Okay?
Now, this is the molecular record that these cells are carrying with them today. We can turn to the fossil record to look for other evidence of this as well.
[new slide posing the same question WHEN did Earth get its molecular oxygen-rich atmosphere?” now with three photos, on of stromatolites on the sea floor – masses of sediment trapped in cyanobacterial mats that are continually growing upwards towards the light – with an inset photo of the top of a stromatolite showing oxygen being released by cyanobacteria in modern day, as well as a photo of a fossil of a Proterozoic stromatolite]
And here are one of the most well-known Precambrian, that is before the – the Protozoic and Archean, the pre-Phanerozoic part of the geologic record. That’s what I mean by Precambrian. These are stromatolites. They’re actually really abundant around Wis – around town here. I’ll show you some. And what they are are just patterns and sediment caused by microbial mats that grow. And these are modern ones. They’re really rare in today’s ocean for reasons that are cool, but Ill show – I’ll talk about briefly in a minute. You’re not likely to see one today. But they stick up out of the sea floor. You know, this thing I can break it off and hold it like this. And they stick up positive like this because they’re basically cemented together with minerals. And on the top surface of this you can see these little bubbles. These are oxygen bubbles being given off during the day by cyanobacteria colonies that are crust – encrusting the surface. And they grow up because the molecular – because the cyanobacteria are trapping sediment and it’s solidifying and they’re kind of pushing their way up to keep in the light and so forth and they kind of grow like this.
Now, these fossils that we find are just patterns in rock that are very distinctive. They don’t actually have cyanobacteria in them, but they are formed in a way that we think cyanobacteria were involved very clearly.
[new slide posing the same WHEN question about Earths molecular oxygen-rich atmosphere, now with two new photos of two different fossils of Proterozoic stromatolites – with the geological timeline still at the bottom of the slide]
And it turns out that in the old part of the rock record, even though you’re never going to see them today, geologists have known for a long time that back here in the Protozoic, they’re everywhere and they’re huge. This is a guy standing here. This is actually in the U.P. of Michigan. And you can see these lines here. These are giant stromatolites. These are like making reefs, basically, in the Precambrian. Okay?
Now, so what’s the physical?
[Shanan Peters]
We have the molecular evidence for cyanobacteria, what’s the physical evidence for having abundant stromatolites or abundant cyanobacterial communities? It’s not enough to have one cell some place evolved in the nook and cranny and one little colony is growing. In order to make a lot of oxygen, it has to be everywhere. The Earth has to be green. And so, we can look at this record to get a sense of how many str – how many of these colonies are there. Are they everywhere? Are they occupying all the marine environments, for example?
To do that, we need to go everywhere and find a lot of stromatolites and document where and when they occur. That’s hard to do when there’s not a database for this, yet. But geologists love writing about these things, it turns out. And so, actually, this is our own Bob Dott and Charlie Byers, retired professors here in the Department of Geoscience.
[slide featuring a photo of a stromatolitic rock formation on the left and a research paper titled, Sequence Stratigraphy of the Lower Ordovician Prairie Du Chien Group on the Wisconsin Arch and in the Michigan Basin by the aforementioned Bob Dott and Charlie Byers on the right]
And they’re actually writing about rocks here in Wisconsin. Actually, these – this is the Shorewood Hills quarry. If you go around there, there’s a little quarry. A lot of you nodding. You probably know the one. Go up and look for stromatolites. You’ll find them there. Okay? And they love writing about them. And here’s the text that says the basal disk has stromatolites. And so, in principle, one could just read all the published literature and not bother to go in the field and find mentions of stromatolites from all over the world and compile them. That would take a lot of time. Even for grad students that would take a lot of time.
[laughter]
[new slide titled, GeoDeepDive machine reading approach with a diagram of the natural language processing that the machine uses on the top and a photo of graduate student Julia Wilcots next to a bulleted list of highlights of the GeoDeepDive machine – it is a digital library containing PDFs of published papers, over 3.5 million papers from all disciplines in Elsevier, Wiley, Taylor & Francis, Canadian Science Publishing and several professional societies and open access providers, it is coupled to high throughput computing facilities at U.W.-Madison, and all documents are parsed and annotated in preparation for machine reading tasks, text and data mining]
A little side story, I’m going to tell you is, I’m involved with the project here on campus. I’m very excited about that’s called GeoDeepDive. It’s a horrible name. It’s actually a digital library that anybody – it’s a campus resource. And it’s actually connected to a high throughput computing system so that we can read millions of scientific papers and actually get information out of them. Not just find them but get information out of them. And that’s what Julia here did. She’s a – actually a native of Madison but didn’t go to school here but worked in the group for a summer, and she did the first analysis of all these papers. In this case, more than three-and-a-half million papers, wrote a computer program to find stromatolites and figure out their ages based on the text. That was done here on campus, and – and we are able to do this in clever ways because the computers read the sentences and parse them into linguistic relationships between words and all this stuff. If you’re interested, I can tell you about it later.
[new slide titled, Prevalence of stromatolites in marine shelf sedimentary rocks, with a graph below it with the geological timeline on the bottom and the number of stromatolite mentions for each geological period on the y-axis showing a large mention of stromatolites in the Proterozoic period]
But here’s the result after doing this for the first time, quantitatively, for all of the published records of stromatolites. And this is a busy graph, but it’s the frequency from – so, if every – if every marine sediment that – that we have was a stromatolite, it would be one. If none have stromatolites, it’s zero. So, it’s normalized for how much is there. And what you’re seeing here is today they are super rare. There’s virtually no marine rocks that have stromatolites. But in the past, going all the way back to 2.5 billion years ago, they are everywhere. And this is a very, very conservative way to measure it. If you look at a more sensible culling, most of the Precambrian is everywhere, stromatolites are everywhere. Okay. So, all the way back to where we think cyanobacteria first appeared, these signatures of cyanobacteria communities are just everywhere on – on the Earth. The communities are in place.
[new slide returning to the question of WHEN did Earth get its molecular oxygen-rich atmosphere? with a photo of a fossil stromatolite and the fact that oxygenic photosynthesis is old and was widespread on the left and the geological timeline still at the bottom of the slide]
Okay, so when did molecular oxygen – when did Earth get this molecular-rich oxygen atmosphere? Well, this happened, this key step happened. The evolution of cyanobacteria, and widespread communities of them, is at least 2.5 billion years old. At least. But that, as we’ve already seen –
[Shanan Peters]
– doesn’t mean there’s a lot of oxygen in the atmosphere. So, we need to look at other proxies for the molecular concentration of – the concentration of molecular oxygen in the atmosphere. And oxygen is convenient for this purpose because when you introduce it into the environment, it – it just reacts with everything. I mean, that’s why I’m able to be all energetic up here giving this talk because I’m powering my body with oxygen burning. It’s very energetic reaction, and it’s happy to react with many things. And so, introducing oxygen to our surface environment changes not just life, it changes the physical environment, radically. And there’s very clear signatures of the perturbation to the geochemical system that is caused by all the sudden having a bunch of molecular oxygen being pumped into the system. And that plays out in all kinds of records we can see in the geologic record.
[slide still posing the question of WHEN Earth got its oxygen-rich atmosphere and the geological timeline at the bottom, but now with two photos – one of branded iron formations and one of detrital pyrite and two diagrams of how these redox-sensitive proxies are created]
There’s a bunch of them. This is – I’m going to go by really quickly here. But this – there’s pyrite, fool’s gold, FeS2. It’s not stable today. Like, if – if you got some pyrite and put it in a glass of water and, you know, shook it around, eventually it would dissolve or it would rust away. Okay? You – you – you’re not going to find pyrite beaches in the world’s oceans because there’s too much oxygen around and it gets destroyed. It turns into rust and – and other things. But if you don’t have oxygen, pyrite’s very stable, and it will actually make beaches. And these are actually layers of fool’s gold sand that showed up uniquely in – in the Earth during the little window of time when there was no significant molecular oxygen in the atmosphere at all.
Now, when that world exists, there’s also other things. Things like iron become soluble, much more soluble in the oceans than they are today because oxygen grabs hold of iron and makes it rust, immediately. But if it doesnt – if oxygen is not around to do that, Fe plus 2 will just be in – available like chlorine in – in sea water.
And there’s these interesting sediments called banded iron formations that your car is quite literally derived from. This is where most of the world’s economic iron deposits come from because there was this time when molecular oxygen’s bubbling into the Earth’s surface environment for the first time –
[Shanan Peters]
– that the Earth’s oceans literally rusted. And these unique sediments show up only during this time, presumably in response to this load of iron that was mopped up by this molecular oxygen and put there forever afterwards. Okay.
So, and then there’s one – theres other more fancy proxies. This sulfur isotopes in the Earth – remember sulfur’s a common volcanic gas, in the presence of oxygen and without oxygen there’s isotopes that fraction in very different ways that show a clear signature of divide between the old record that has no molecular oxygen, in which case these – this fractionation is very visible, and after the time when we have molecular oxygen in the atmosphere, at which case we just lose that signature in the sulfur isotope record. So, there are other – a variety of different proxy records that say this interval right here, this band between 2 and 2.5 is changing radically in the molecular oxygen concentration of the atmosphere.
[the previous slide animates on two more photos – one of red beds Fe2O3 and the other of Gypsum CaSO4]
After that we see things that tell us that there’s plenty of oxygen around. That includes red beds. So, soils that are red. You’ve probably seen them if you’ve paid attention. They’re red because of rust in them. The oxygen latches on to iron minerals that are liberated during chemical weathering, and it rusts the soils. Those show up for the first time. Other things, like gypsum, you may not think about it but this – gypsum, drywall, that’s a signature of an oxidated Earth. It – the sulfate in there is sulfur that has been oxidized, consumed molecular oxygen in the atmosphere, put in the ocean as sulfate, and we get it back as gypsum. Okay? That shows up in the rock record very late and tells us, okay, there’s a lot of oxygen in the atmosphere, enough to make gypsum.
The other major record we have for the history of atmospheric oxygen of course is biology.
[new slide with the geological timeline at the bottom and the question of WHEN at the top and three new photos are superimposed on top of the previous photos, titled biological proxies, one of a prokaryote, one of a Eukaryote, and one of metazoans]
Organisms care deeply about the concentration of oxygen in the atmosphere, and there’s sort of three main modes of life reflected in these three time periods for which named after life, not surprisingly, where we have a world that’s dominated by prokaryotes, like cyanobacteria, and then eukaryotes. And, actually, this specimen here is a little eukaryotic alga, and it’s actually, it’s about 2.1 billion years old, and it’s found just up the road in the U.P. of Michigan in the Negaunee iron formation. But it’s, you know, big eukaryotic cells show up at about 2.1 billion years. Then there’s a long period of time where not much happens, and then we get the Cambrian explosion and the, you know, the wonderful, fast diversification of animal life at the end of the game. Okay?
Combining all of these different proxy records together –
[new slide titled, Great Oxidation Event GOE, with a graph of geological time at the bottom and level of oxygen on the y-axis, showing low levels of oxygen in the Archean period (bacteria, archaea), then the Great Oxidation Event in the early Proterozoic leading to single-celled eukaryotes, and finally and then large amounts of oxygen in the Phanerozoic coinciding with animals]
– we’ve arrived at sort of this canonical model for the history of molecular oxygen concentration in the Earth’s atmosphere. Again, this is not my work. This is synthesis work from all these different sources.
Now, we know there’s this time when there’s virtually no oxygen in the atmosphere for all those proxy records like the mass independent sulfur fractionation I talked about before. We know cyanobacteria weren’t probably around until somewhere in this pretty long but still, you know, this – this time frame. And then we have evidence that oxygen is being produced and released in the surface environment. Now, there’s a lot of interesting debate about whether or not oxygen rise – rose really quickly here, up to near modern levels, this line up at the top, and then fell, but what we do agree on –
[slide animates on a pink line indicating the predicted rise in oxygen levels after the Great Oxidation Event showing steady oxygen levels until the introduction of animals and then indicating perhaps a rise in oxygen levels above the modern level of atmospheric oxygen early in the Phanerozoic period]
– is that sometime after that initial what’s call the great oxidation event where we cross this divide between no oxygen and at least some molecular oxygen happened around here. And then there was a long period of time, most of Earth’s history, a good billion years or so, often called the Boring Billion, before oxygen rose rather precipitously into the Phanerozoic.
And so, one really important question is not to say, is – is really why is there this long delay?
[the words Why this long delay?? animates on the slide over the period of the long delay on the graph]
Oxygen was being produced. Geology was happening. The world was doing its thing. Why was this long delay between the onset of oxygenic photosynthesis and the buildup of oxygen in the atmosphere? That’s really the question.
Now, I hoped I’ve convinced you that, you know, these things must be part of the answer, right? Rocks.
[Shanan Peters]
There’s something about the rocks. And so, maybe the answer is encoded in the burial of organic carbon that’s in rocks. Seems reasonable.
[slide titled, Answer must be in rock record via burial of organic carbon, featuring a photo of a fossil in a rock bed]
[new photo of large piles of rocks that have been eroded away from a mountain in the Andes]
But here’s the rub. Im – I’ll cut to the chase and tell you that is correct, but it took us, as geologists in a discipline – science, a while to figure this out, I think. And some people will still disagree with me just to be clear about the record. But I’m going to make the case that we, kind of, missed – missed the picture here a little bit. And this is why. This is – this is geology in your face, right? This is the Andes Mountains. I got back from a wonderful alumni-supported field trip there with a group that’s working in the Andes, and it was fantastic, in-your-face geology. Erosion is like the thing you’re struck by in the Andes. Volcanism and erosion. Just in your face, everywhere. There’s rocks, they’re – theyre forming new rocks by erosion, and they’re being destroyed. And geologists have looked at these things, the rock record –
[slide animates on the book cover for The Origin of the Species and the Chapter heading for Chapter Nine – On the Imperfection of the Geological Record as well as a profile photo of Charles Darwin with his quote – I look at the natural geological record as a history of the world imperfectly kept.]
– in a way that is traceable all the way back to this guy, Charles Darwin, who is of course a geologist, right? He was well-known at first for being a geologist and then secondarily become well-known for his contributions to biology, which were many.
And in “The Origin of Species,” definitely one of the most influential scientific texts ever written, he has a chapter titled, well, not very subtly, “On the Imperfection of the Geological Record.” And in this chapter, he says, “I look at the natural geological record as a history of the world imperfectly kept. It’s written in a changing dialect, and of this history we have just the last volume. And of the last volume, the last few chapters. And of those chapters, a few lines.” And so on. And he – he portrays the geological record as this tattered manuscript upon which the history of life, in particular, but Earth, in general, was written, but then it has been ravaged by time and geology to render it not useful, ultimately, in his argument, for really telling us about the history of life, mainly because he had peculiar ideas about what he thought he would expect to see in the fossil record, I guess.
Now, what is good about this hypothesis is it makes very clear predictions.
[new slide with an animation of a backhoe digging up rock along with a circle that has strata of rocks – one being sedimentary rock (S) and the age of those sediments being S(t) where t is time]
And this is basically the prediction. Here’s a little cartoon of it. Erosion happens and erosion makes sediment from rocks, and – and those rocks – that sediment gets put back into the rock record, and this giant thing just turns, and its slowly going away, and if we watch it over time, so this – nothing ever changes because it’s a cycle, and if we watched it over time, there’s less and less and less and less of a given age as we go backwards. And, mathematically –
[slide animates on the book spine for On the Origin of Species in the lower left and the equation S(t)=S0e-rt – the age of the sediment is a function of how much sediment there was in the beginning times the exponential decay function]
– this is really the prediction. That is the amount of rock that we have at any – of – of time, “t, say 500 million years ago, is a function of how much we started with times an exponential decay function, the decay curve. Okay? This is the formal prediction from Darwin’s description of the geological record. It also, by the way, is wonderfully Lyellian and Huttonian. This uniformitarian view of the world where nothing changes. It’s a giant cycle. Things come in, things come out, nothing goes anywhere. In fact, Hutton took it to the extreme to think that the Earth had no beginning.
[Shanan Peters]
Everything was cyclical and so on.
Now, this is a prediction but getting the data together to test this was very difficult and it was like, what, I can do the math, I promise, 110 or 120 years before we had any attempt to actually test this hypothesis. Okay?
And this is actually it. 1970 Nature paper.
[slide with an excerpt of the Nature article with the headline Denuding of the Continents and a graph from the article to its right]
It’s hard to see the highlights I put here, but in reading this, I love this stuff. This is a very clever thing. This was done to address the denudation of the continents. How fast the continents are going. And just for fun and completeness, this person concludes that “man’s intervention has increased the overall denudation rate of the land from 9 to 24 billion tons a year. In this communication, I attempt to verify this pre-human estimate and to defend its general applicability. This was motivated in – in 1970 to try to figure out how important humans are moving around sediment on the Earth relative to geology. I thought it was pretty cool.
But this is the result over here. These are how much rock we have of given ages, going back here. The details aren’t important. What is important is that there were virtually no data. Getting these data – data together is really hard. You have to compile syntheses of geological knowledge that are difficult to assemble. You have to get a big picture view of how the upper crust is put together. It’s not easy. And the only actual data for this analysis were from a Russian stratigrapher right through here, and all this was a – a mishmash, okay, of extrapolation, basically.
However, this fit the notion very clearly that geologists had in mind that the Earth’s crust must cycle continuously and uniformly like this –
[new slide with a different scientific journal article, Controls of Atmospheric O2 and CO2, Past, Present, and Future, with a graph from the article on the right and a photomontage of the circular sediment from the previous slide on the left]
– and this and other analyses cemented the notion. It was a done deal. And so here is a 1976 paper, Controls of- now, everybody knew this thing, this wheel that’s turning slowly, constantly. It’s huge. Right? It’s really big. But it’s turning slowly and constantly. And here’s the heres the – here is this abstract. Geochemical models of the Earth’s surface environment, focusing on O2 and CO2, suggest a dynamic steady state system exists, and that steady state is nothing changes and, if it does, something else has to happen to the system. It has to be perturbed biologically or by some other forcing mechanism. The geology process by itself can’t do it.
[new slide posing the question, Does sedimentary quantity decline exponentially with increasing age?]
So, what I’m going to do is to ask a really simple question: Does sediment quantity decline exponentially with increasing age or not? We, kind of, need to know the answer to this question, like, for real.
[new slide featuring a photo of the Macrostrat database, which is a map of the world with small dots indicating rock units from 1,474 regions]
So, the data set I put together to do this is called Macrostrat. I – I’m proud of it, but I’m not going to say much, I promise. But basically, what it is, is – is a big compilation of basically what you would get if you took a cookie cutter punch through the crust and looked at the rocks and identified their type and age, that’s what it is. And – and so, these little points in the deep sea, we’re going to show results from the deep sea and this is the last time you’re going to see this map. There’s a bunch of them spread around the deep sea. And we’re going to really focus on this North America-centric view of the world, and I don’t have time to go into the reasons why I can show that this is a good proxy for continental crust globally. It’s a large enough sample that’s diverse enough tectonically to be representative of crust everywhere, and I’m not the only one to have found this. But I’m going to gloss over that part going forward.
[new slide titled, Basic Macrostrat column organization, featuring a map of North America with different types of sediment color-coded and placed on a timeline and the bullet points that – they are regionally composited chronostratigraphic columns; surface and subsurface, they are of lithostratigraphic unit-scale resolution in most cases; basic lithology information, 8,155 lithostratigraphic names have been applied to units, full nomenclature hierarchy; 36 thousand names, all rock units acquire continuous time age model based superposition and correlations to chronostratigraphic bins, and they are integrated with bedrock geologic maps]
So, we’re going to zoom into North America here and look at this in a little bit more detail just to have a sense of where the data are coming from. Here it is. This is a little column here. This is the Exshaw column. They all have little names and stuff. But, and, obviously, you can see that this is an extrapolation around control points. That part’s also not important. But what is important here, I’ve cut off the Phanerozoic, so this is the Cambrian. The Phanerozoic portion lasts 541 million years. And the white chunks here are gaps, are gaps in time. Okay? So, there’s no rock of – of this age at this column. The colors are chunks of time here that have rock. And the color of the rock is telling us what type of rock it is. Okay?
At its fundamental level, this is what this database consists of. Now, the first thing I want to make abundantly clear, is when – if – if you pay attention to Darwin or if you’ve read about this, you’ll recognize that Darwin also described the geological record, not just in terms of getting worse with age, but also being gappy. And the gappiness of the rock record was of concern to him.
[Shanan Peters]
He was absolutely right there. There’s lots of gaps, and the vast majority of the time at any given place tends to be occupied by gap. Okay? But music is the space between the notes, as Debussy said, so we’re going to see why that’s a signature of the process we’re trying to get at.
Okay, so, that’s what these columns look like. They’re regionally composited. They include, importantly, the subsurface too. So down as deep as we go, typically. That’s really important, and there’s a whole bunch of data acquired that are, sort of, linked to these rock types and stuff that we’re going to depend on later.
Yes, we’ve made a mobile app. I’m also very proud – proud of this. It’s free. Android and iOS.
[slide with an advertisement for the departments mobile app, Rockd]
You can download the thing. Wisconsin Public Radio has a nice little interview, if you want to dig it up and hear my view of what it is. I’ll let you do that later. But if – if you turn it on, it just gives you, like, our best guess of what you’re standing on, geologically. Okay? So, hopefully it’ll be fun, and it gives you really good maps and you can make observations and stuff. So, check it out.
[new slide showing the interface of the Rockd app with photos of users interactions]
And then, theres an interactive – once you do that – you – youre – if you make your stuff public, you can explore it around in an archive like this. It’s for nerds like me, really, but if you’re interested in just being out in the field and learning about rocks around you, this will help you, trust me. Check it out.
[return to the slide asking the question, Does sedimentary quantity decline exponentially with increasing age?]
Okay, so, let’s get to it. Ask the question, with these data, does sediment quantity decline with age or not?
[new slide with two illustrations of North America, one color-coded map of showing the prevalence of the various sediments and one black and white map with the equation – columns with sediment divided by the total columns giving the answer .26 covering 7.9 million square kilometers]
What I’m showing is one, over here, is just one time slice. Okay? One time slice through the stack of geology. And where there’s color, or solid color in this panel or color here, there’s rocks of that type, that type doesn’t matter for us, but there’s rocks there of that age. Okay? Where there’s white there are no rocks of that age. Okay, so those are the hiatuses. And so, what I’m going to show you are a bunch of measurements of quantity measured in this way. The simplest way is to just simply measure the proportion of columns that have sediment, the fraction, the proportion coverage of sediment on the continent. How many columns have sediment divided by how many columns are there. Easy. Or we could measure it in square kilometers or something like that. Either one. I’ll show you both. Okay.
Now, before I show you the boring time series, which will put you to sleep but I’ll try not to do that, here’s a little animation –
[a digital player moves along the timeline on the bottom of the slide and as it does so the map and the color-coded sections of the map change for the various sedimentary locations that exist at that particular time on the timeline]
– that shows qualitatively what we’re going to do. From one – only from one billion years up to the – up to the present. Okay, and it’s going by really quickly here. But I’ll nar-narrate it. So, for a billion years, up to the – this is the start of the Phanerozoic right there. Now, remember, this isn’t fossils. This isn’t diversity of life. This is just rock. Okay? Just rock. And so, you can see that something changes right about there pretty dramatically. And we’re going to see evidence for that quantitatively in a second, but I’m going to be showing you time series that summarize this madness in numbers. And here it is.
[new slide titled, History of sedimentary abundance – all sediments, with a graph with geological time on the x-axis (starting with 2500 thousand years ago) and proportion of sedimentary coverage on the y-axis showing a large spike during the Paleozoic era, a small dip from the spike in the Mesozoic era and a return to early Paleozoic numbers currently]
Okay? This is now going – so, we saw that animation from here, and so that big jump was that. That you saw visually. And we’re going to be going all the way back to two-and-a-half billion years, typically. Okay.
[Shanan Peters]
Now, before we talk about this long period of nothing in then all the sudden a huge increase, before we talk about that and why looks completely different than most of Earth’s history from, say, 600 million years ago all the way back, we want to as the question, is the Precambrian just bad somehow? Like, do we just not know it very well? And the answer is sort of. We can see some evidence that this is true. Now, it’s a little subtle here, but bear with me.
[the History of sedimentary abundance – all sediments slide animates on three new graphs to the right of the current graphs now of sedimentary package duration, thickness, and accumulation rate. Additionally, on the main graph along the long line where nothing seems to be happening the statement – less robust age control, harder to recognize gaps, animates on]
These packages of sediment at each column, okay, that we saw, those little chunks of color, and what we’re doing here is measuring in the Phanerozoic, in blue, and in the Precambrian, in red, and we’re measuring the duration in millions of years of those packages. And we can see that they’re different. The Precambrian is longer in duration than – than the Phanerozoic.
Now, the reason for this is really simple. In the Phanerozoic we have evolution of animals happening, and we use that to tell time. We’re able to see evidence for missing time in rocks that is hard to see except through the signature provided to us by evolution. And so, in the Precambrian we – we miss those things and we assume that the record is more continuous than it is, and so my columns don’t break out packages like they do in the Phanerozoic. And we can see that here quantitatively. However, the Precambrian is also thicker in meters. Okay? So, there’s more rock in a little longer chunk of time, and if we take the combination of the two, it’s what’s really important for today, is the rate of sedimentation, accumulation rate, meters per million years. The two distributions collapse. Okay, so take my word for it that there’s no evidence that there’s something fundamentally broken about the Precambrian record here. Okay? So, this signature –
[return to the main graph of the History of sedimentary abundance – all sediments]
– is largely real. Okay?
So, let’s ask the question, is this exponential? No. Right?
[an exponential equation P(t)=0.16e-times ten to the .0009t and t1/2= 770 Myr as well as an exponential curve is superimposed over the top of the graph showing the results are not exponential]
We can fit and exponential curve to this. Force one. We can fit an exponential to anything. It doesn’t mean it’s a good description of the data, and this is a horrible description of the data. And it gets a very long half-life, but that’s not important. It’s just a bad description –
[two pink bars animate onto the graph one at the Great Oxidation Event and one at present day showing a decreasing quantity of sedimentary coverage]
– and why is it a bad description? It’s because the exponential models predicated on this notion that once you have a package of rock –
[Shanan Peters]
– it has a one-way street out of the system, and its – as long as that’s happening approximately constantly, you’ll get an exponential decline. Decline should be the signature. Actually, there’s only two intervals of time that show any decline at all as a function of increasing age. Right here decrease –
[return to the previous slide]
– and then a whole bunch of up and down but no decrease over long time and then another decrease here. All of the net decrease in quantity with increasing age happens in just those two little bars, which is remarkable. And right away not what Darwin would have expected.
[the graph of the History of sedimentary abundance – all sediments now animates on green bars for the rest of the graph]
The rest of the time is these intervals where it’s going up and down, up and down but not going anywhere. Not going up, not going down. It’s a state of oscillation back and forth around some average. And that’s what the rock record – and thats why exponential is a horrible fit.
[new slide titled, Plate tectonics results in crustal differentiation, showing an illustration of plate tectonics for continental crust and oceanic crust and noting that continental crust is buoyant, high-standing and old, while oceanic crust is less buoyant, low-standing, and constantly recycled]
Now, I’m going to speed up here, but everybody has heard of plate tectonics. There’s ocean crust that goes away. There’s continental crust. You’re standing on it. And it’s old because it never goes down, the continental crust. Okay, so there’s a huge bimodal distribution in the ocean floor and the continents that showed up with the elevation that we talked about, and it shows up tectonically in survivorship. Okay?
[new slide titled, Continents have both exposed and submerged areas, featuring a satellite image of the southern part of the North American continent showing the continental crust both as a land mass and as partially submerged along the east coast and Florida and then dropping off to oceanic crust and noting that submerged continental crust has low elevation with accumulating sediments while exposed continental crust has higher elevation and supplying sediments]
Now, let’s go back up onto the continents. There’s some parts of the continents that are flooded with shallow seas, like off the coast of Florida. This little ramp here you can see on Google Earth is shallow water because it’s on continental crust. And this big drop – boom! – down to the bottom, that’s oceanic crust. Okay, so some parts of the continents are flooded with seas, which means sediment is accumulating in them. And some parts of the continent, like Wisconsin, are not and sediments are going down the river.
There’s these different domains of sediment. We can do a better job of parsing out sediments into different domains –
[new slide titled, History of sedimentary abundance – deep sea (non-marine, shallow marine), featuring a color-coded map of the globe showing the differing ages of the Oceanic Lithosphere along with photo of the deep-sea floor and a graph of deep-sea sedimentary abundance over the geological timeline showing a rise in abundance starting in the early Mesozoic period]
– and that’s what I’m going to show you here because it’s sensible geologically.
So, we’re going to take that curve we saw before and we’re going to decompose it into deep sea, non-marine, which is the sediment up above sea level on continents, and marine on continents. Here’s the deep-sea record. This is the record of, measured in this same way, proportion of coverage, just like before, and it drops down precipitously just like we would have expected from the beginning and Darwin’s point of view. And the reason for that is the sea floors are young, they’re constantly being destroyed, the oldest sea floor on Earth is around there. That’s still in place. Okay?
[new slide featuring just the graph of the deep-sea sedimentary abundance with an inset of the data and a graph just starting with the early Mesozoic period (oldest sea floor) to present day. The slide also shows the exponential equation for this data and an exponential curve superimposed on this graph showing that this deep-sea data is indeed exponential]
If we expand this up a little bit, it fits the exponential pretty well. That’s a good mathematical description with oscillations that are cool but a subject for another time. Where we have a clear signature of formation and destruction, we see a clear signature of formation and destruction of sediments, just like Darwin would have guessed.
[new slide titled, History of sedimentary abundance – non-marine, featuring a graph of non-marine sedimentary coverage over the geological timeline showing a mostly straight line until the Paleozoic period whereupon sedimental coverage increases but in peaks and valleys. Additionally, the slide features four inset photos – one and illustration of North America, a photo of a river winding across a countryside, the photo of the erosion in the Andes shown previously, and a photo of a rocky shoreline]
Now, how about the non-marine record. Now – now, the non-marine record is again an in-your-face record. We see erosion happening all around us. Well, that’s because were – we live above the ocean. We’d have a different perspective of the Earth if we were fish. But erosion is very heterogeneous on land. We have rivers at high elevation and low elevation and so on, and this is the total number, again, proportion coverage of sediments on the continents, and it drops precipitously back through time, but it sticks around forever down here.
[new slide now with just the graph of non-marine sedimentary coverage over the geological timeline and again with an inset graph of the same data just starting in the Paleozoic to the present and showing the exponential equation for this data and the exponential curve superimposed over this data showing that indeed this data is also exponential]
And so, if we zoom in here just on the past, sort of, 400 million years or so, it’s, sort of, okay exponential fit. Not very good, but not a passable exponential fit to the data.
[Shanan Peters]
This makes sense because unlike the sea floor, which all undergoing sea floor spreading and subduction, non-marine sediments are everything. Theyre – theyre – they include glacial sediments dropped unceremoniously from Canada by ice on Wisconsin that’s slowly eroding away. That’s non-marine sediment. The sediment in Lake Monona, it’s great – great record but not very long and it won’t be here forever. And so on. It’s just a really heterogeneous mix of things, and you’re mixing these things together and measuring one exponential fit, and that’s like measuring the half-life of the atoms. It doesn’t make any sense. Some stick around a very long time, some don’t, some never go away. They have – and we’re mixing them together here. So, but still, we see a very clear signature of there’s loss because these sediments are deposited in the place where that loss is happening.
How about marine sediments? So, marine sediments have no such trend of decreasing quantity with increasing age. In fact, in North America, there’s way more Cambrian sediment, we’re standing on it, by the way, than there is of recent marine sediment. And so –
[slide titled, History of sedimentary abundance – shallow marine, featuring a graph of proportion of shallow marine sedimental coverage over the geological timeline that is a graph that is almost identical to the all-sediments graph and is clearly non-exponential]
– obviously, and exponential fit to this does nothing. It has no explanatory power at all. So, what is controlling the history of marine sedimentation on continents, the rocks of Madison. Bascom Hall is built on marine sediment, not through non-marine sediment, not deep-sea sediment, marine sediment put here 500 million years ago by seas.
[new slide under the heading of history of sedimentary abundance – shallow marine now with a graph plotting the area of sediments over geological time showing large peaks and valleys over time and noting that it is tempting but perhaps premature to relate these cycles to current hypotheses of sea floor spreading and plate tectonics]
This signature records tectonics. Okay? It records tectonics. It records the – this is that same, in the Phanerozoic now measured as area, rise and – and – and fall and rise and fall again, often called the M curve. This is the super continent break up coalescence, break up and coalescence cycle. That’s the quantitative thing that’s pushing the seas around on the continents.
[new slide with two side-by-side illustrations, one of the North American continent during the Pliocene era 5 million years ago, looking mostly like it does today, and one of the North American continent during the Late Cambrian era where most of what is North American now was under the sea]
And, basically, the world is going back and forth between one that looks like this, more or less like today, to one that is like this, like the rocks that Shorewood Hills quarry were deposited in, a shallow sea that lapped all the way up to Wisconsin. And that state is changing, and that state is –
[return to the History of sedimentary abundance – all sediments graph with the green and red color coding now showing the area between the two red declines as the era of continental flooding]
– what’s giving you these wild oscillations in the Phanerozoic. Okay? So, this green part here, these changes are caused by continental flooding changes which are caused by mostly tectonics, a little bit of climate, and that’s what’s driving it. And I would argue that’s what’s also driving this. This decline here –
[points to red decline bar near the current era]
– is driven by deep sea, non-marine, deep sea sediment and non-marine sediment. What about this – this one?
[points to the first red decline bar on the graph in the Neoproterozoic era]
This is the only other decrease we have. Maybe this is caused by erosion. Well, let’s go look at it.
[return to the shallow marine sedimentary abundance graph]
This is the non, again, the shallow marine record. That big decrease at the start of the Phanerozoic is a marine sediment signature on the continents. Let’s go look at it in the field.
[a photo of a section of sediment from Colorado animates on the graph as an inset showing a Precambrian crystalline basement on the bottom and Cambrian marine sediment at the top with a dashed line between the two marked G. Un. showing the area that corresponds to the early decline on the sedimentary abundance graph]
Wisconsin’s actually a reasonably good place to do it. This one is from Colorado. And this is pretty typical of this boundary right here. This is Cambrian marine sediment deposited on top of granite. Very old granite, in this case a billion-year-old granite.
[the inset photo from Colorado is replaced by a photograph of the Grand Canyon]
The Grand Canyon has at its base a surface that John Wesley Powell called the great unconformity. And you can see it from the rim of the canyon. It’s one mile of Phanerozoic sediment, starting of that age right there, sitting on top of metamorphic basement rocks that are 1.7 billion years old. Okay, so this decline here in sediment quantity is not a decline at all.
[the first red bar of decrease is animated off the graph and replaced with a green bar showing an increase and labeled as initial flooding of continents]
It’s actually an increase in flooding across the continents that were once exposed and eroding. And the oceans covered them and put sediments there, and that sediment is still there to this day. Okay, so that actually records tectonic state change –
[Shanan Peters]
– across the boundary. What about this? A mystery for another time.
Okay, so we have three different components. Deep sea sediments that have clear signature of cycling in them, just like Darwin predicted because that’s the world that he described.
[slide titled, History of sedimentary abundance – all sediments, now featuring all three previous graphs, deep-sea, non-marine, and shallow marine all on one slide]
Non-marine sediments, very close to what Darwin described because he’s walking around on it, probably impressed by it in the Andes just like I was. And shallow marine sediments, which have none of that signature at all. Instead, they have a process signature driven by tectonics and changes in state of the world. And, it turns out, that 75% of the volume of sediment we have is that stuff. Okay?
[return to the slide posing the question, Does sediment quantity decline exponentially with age?]
So, the answer to the question: “Does sediment quantity decline exponentially with increasing age –
[return to the slide featuring the graph of all sediment coverage over geological time, with the answer NO! and the statement Net sedimentation reflects major changes in how much was sequestered on continents]
– is no.” It does not. Okay?
Now, this history, if you’ve been paying attention the whole way through and – and are easily pasting things together in your mind, this should look –
[new slide superimposing the graph of atmospheric oxygen over the top of the sedimentary graph showing a strong correlation between the two]
– very familiar to that proxy history for atmospheric oxygen and life that we saw before. Prokaryotes, eukaryotes, metazoans. And this curve for the metazoans in the Phanerozoic is – this is actually what it looks like on a relative scale. The history of atmospheric oxygen, in red here. The canonical history, maybe it went up to one or maybe not, but a long time or it didn’t do anything and an abrupt rise in the Phanerozoic and then wiggles around in the Phanerozoic. In black now is the data we just saw on a logarithmic scale for the amount of sediment on continental crust. And it, at the sort of pattern level, looks very similar to the history of atmospheric oxygen. A long period of not much going on, an abrupt rise, oscillations in the Phanerozoic. Now, we can do better than just say, Well, look, it’s similar, good enough, and go home –
[slide animates on an equation of the carbon burial model]
– because we can actually link this and test the hypothesis that there’s a direct causal connection by calibrating a carbon burial model that is the ultimate source of oxygen in the system. And so, the change in mass of oxygen in the atmosphere as a function of time is – is equal to the total amount of organic carbon we’re bearing. The mass of it. Molar ratio, one to one. One mole of carbon goes in, one mole of oxygen – molecular oxygen comes out. Now, here’s the rub. That oxygen can be consumed, so there’s a negative sign here, that can be consumed by organic carbon. We are doing it right now when you go home and turn on your car, you’re going to be doing this reaction part right here. And that reaction proceeds as – at a rate that’s related to how much we have around, the amount of organic carbon that’s actually in the crust. You can think of it as, like, the charge capacitor. How much that is, weakly dependent on the concentration atmosphere oxygen and a constant. And then this term is really important. It turns out that oxygen reacts with everything. Well, not everything, but a lot of things in the Earth’s crust itself. That’s what this term is. Okay?
Now, the first order of business is to calibrate this – this – this – Forg. How much carbon is going into the crust and when?
[new slide titled, History of sedimentary abundance OC burial, posing the question – How much O2 has the surviving rock record sourced to the surface? Additionally, there are three graphs, two of sedimentary volume flux, and one of organic carbon flux]
And to make a long story short, really quickly, this is what you need to do it. This is now the same data but turned into a volume flux. Okay? This is in cubic kilometers per million years. This is now the weight percent of organic carbon. How black the rocks are, on average, basically? And this is empirically calibrated with data. I don’t have time to tell you about it, but trust me, it’s a good estimate for how much organic carbon is in rocks. And then this is the combination of these two to get you the burial flux. The organic carbon burial flux in moles per million years. Okay?
Now, here’s a question for you: how much O2 has the surviving rock record, the one that we can point to and say, “Yep, there it is.” How much is that sourced to the Earth’s surface environment? So, here’s part of it right here. Right? How much total oxygen is in that thing?
[slide animates on the answer to the question – approximately 35 times the oxygen of the present-day atmosphere]
It’s about 35 times the present atmospheric oxygen concentration. 35. If you put that ox-oxygen in the atmosphere right now in a bottle, 35 times that would be what the geological record has stuffed into the Earth’s surface environment. The reason we don’t have 35 atmospheres worth of oxygen is because rust happens. Other exit channels for oxygen. Your car is doing its job right now rusting away a little bit in the rain.
[the slide animates on the carbon burial model equation – dM divided by dt is equal to Forg (Macrostrat) minus k1M (k1=Iron oxidation) minus K2 (S.C. Oxidation) times Borg (Macrostrat) times the square root of M]
Okay, so the way we do this model is we measure that. That’s this thing. This – the “k” constant we – we – or this constant we take directly from an estimate of oxidation of iron in the crust. This one – this decay constant – or this constant we take directly from estimates of organic carbon weathering in crust, and we combine them together to make a model, forward model, calibrated by the actual record for oxygen, this is what we get.
[new slide titled, History of atmospheric oxygen – Macrostrat model, featuring a graph with four variables plotted over the geological timeline, variable TOC, invariant TOC, full model space, and canonical history and all four seem to correspond to the earlier sedimentary graph]
Now, for convenience sake, in gray, you can barely see it here, is the canonical model. The full model space – now, there’s different ways to assign carbon values and so forth.
[Shanan Peters]
The details of – of how we do that are subsumed as error envelopes around this estimate here. And so, there’s two different models of how to distribute TOC, and the whole model space, including some Monte Carlo simulations, is shown by this red curve here. And so, this is, on an absolute scale now, the fraction relative to the modern atmosphere of oxygen. And we see a whole bunch of things that look just like the empirical proxy records, including a delayed Phanerozoic rise. For the nerds in the audience, it actually turns out –
[return to the previous slide now pointing out the delayed Phanerozoic rise and the Permotriassic drop]
– the Cambrian explosion happened when this first rise started to go up, but the rise of oxygen to modern levels was delayed even further. It didn’t really get to modern levels until probably 10 million – into the Devonian, probably. And then a big drop at the Permotriassic, the largest mass extinction in the Phanerozoic, right there.
[slide animates on the early transients in the Archean era and the sustained low in the early Proterozoic era]
The other thing that we see is the sustained low. This is probably the most impressive result, honestly, this transition and this long state. And we see these early possibilities of transient whiffs. Now, of course, down here it depends on if that’s photosynthetic carbon or not.
[return to the slide titled, History of sedimentary abundance – continental crust, showing a graph of fraction sedimentary coverage over geological time along with the graph of atmospheric oxygen superimposed and the statement – Oxygenation of Earths atmosphere is dependent on biological evolution of photosynthesis, but was controlled by a geodynamic process]
Okay, so the oxygenation, the timing and magnitude of oxygenation of the Earth’s atmosphere. Yes, it depends on biological evolution, but biology has been doing its thing forever, basically. The timing of the oxidation of Earth’s atmosphere to get to a place where we can have life like us is dependent entirely on geology. It’s – its determined by a geodynamic process that turned on and suddenly allowed the crust to store fossil fuels, basically.
[return to the continental sediments graph by total coverage]
Now this doesnt mean erosion – I’ve done – Ive gone out of my way to argue that erosion is not a thing. But there’s clearly still erosion happening, right? No question about it. And if you were forced to come up with a model for it, that would be it. And – and, you know, it has a really long life.
[return to the continental sediments graph now by area]
Now, I’m going to – Im just going to just emphasize something here.
[flipping back and forth between the two graphs]
See this little positive residual here? It’s positive this whole stretch. Most of the time it’s negative, and so there’s a pretty pronounced positive residual here. I’m going to re-express this axis set instead of proportion coverage – area. It’s the same data. It’s just that there’s non-random distribution of areas. That’s all it means. But you can see this bump gets bigger. And now we’re going to fit an exponential to it here. And you can see that positive residual in the same way there. Okay? Now, I – this is very slow erosion, right? The half-life is 1.2 billion years. I mean, it’s basically not doing anything at the – at the Phanerozoic scale. But, still, this rock is two billion years old. It’s a couple half-lives. And so, we can undo the effects of erosion mathematically, and this is what we get.
[new line is drawn on the continental sediments graph by area representing the graph if erosion is taken into account – now with a large spike in the Paleoproterozoic that is a Phanerozoic-like mass showing that there was an abundance of organic carbon at that period]
And this is the moment where everything should come together, and you’re like, “Oh, wait a second.” This suggests that maybe we accumulated a Phanerozoic-like mass of organic carbon in Earth’s crust for geodynamic reasons similar to this one but then it stopped. And we lost it.
Now, if we take this as our calibration for the organic carbon burial history and we use that to predict what atmospheric oxygen would have done, it looks like this –
[new slide titled, Possible Early Rise in Atmospheric O2?, featuring a graph of the fraction of PAL oxygen over geological time showing a spike in oxygen-rich atmosphere in the early Proterozoic that declines halfway through the era to almost negligible until it bounces back again in the Phanerozoic and present day]
– using the same rules. And what we find, as some people have suggested based on proxy records, shockingly, that atmospheric oxygen may have gotten to near modern levels but not stuck around. Which means it may not stick around in the future.
Now, if you think about this for a second, it should be really obvious because burying – let’s say we reach this hypothetical steady state that Darwin so wants, and geologists still somehow cling to. If that’s the case, that means organic carbon is being buried and oxygen is being sourced, but that oxygen is being consumed by organic carbon that’s being removed and weathered from the system. And so, you are at steady state and there is no flux of oxygen into the atmosphere. What’s being produced is consumed.
[Shanan Peters]
And that would be fine except for the problem of this term right here. Your car, basically. There are other exit channels for oxygen from the system. And it’s not sufficient to have organic carbon buried that’s produced by photosynthesis. You have to bury more of it and more of it and more of it so that the mass in the crust is continuously growing. That’s the only way to maintain an atmosphere that has oxygen in it, and that is definitely dependent on geodynamics.
Now, within the Phanerozoic, a transient hiccup in the continent’s ability to do this happened. Right here at the end of the Permian. That was the largest mass extinction in the Phanerozoic. A transient interruption. In fact, geologists have called this the coal gap. And there’s all kinds of reasons for the coal gap, but it had a big impact on oxygen in the atmosphere. We got out of it because, well, you might remember the power plant over here that used to haul in coal – coal. When I first got here, they were still doing this, and they converted it. That coal came from rocks of this age. There’s a huge amount of Cretaceous and Paleogene coal, young coal, that rescued the Earth from this state. It grew the mass again.
Now, this suggests that it’s not sufficient to have oxygenic photosynthesis through this cool enzyme called Rubisco or something like it on another planet. It’s not even sufficient to have plate tectonics. It – it has to be combined in a way that allows you to continually go this mass, and an oxygen-rich atmosphere may not be permanent anywhere.
So, I’ll end with just the – the main point I want you to take home from this –
[slide featuring a view of the Earth from space and superimposed on the image is a circular diagram of the interconnectedness of the Solid Earth at the top, the Rock Record in the middle and the Fluid Earth on the left edge and the Living Earth on the right edge]
– and that is the Darwinian view of the rock record, this incomplete, tattered manuscript is really missing the point. It has an archive of climate, and it has an archive of life, and it has an archive of tectonics in it. We can read the history of Earth through that manuscript pretty well.
It turns out, however, that the formation of this manuscript is the thing that’s driving the entire system. It is an active participant in the evolving Earth system. Without the sedimentary record, there is not animal life on a planet anywhere.
And I’ll leave you with that.
Thanks.
[applause]
Search University Place Episodes
Related Stories from PBS Wisconsin's Blog

Donate to sign up. Activate and sign in to Passport. It's that easy to help PBS Wisconsin serve your community through media that educates, inspires, and entertains.
Make your membership gift today
Only for new users: Activate Passport using your code or email address
Already a member?
Look up my account
Need some help? Go to FAQ or visit PBS Passport Help
Need help accessing PBS Wisconsin anywhere?
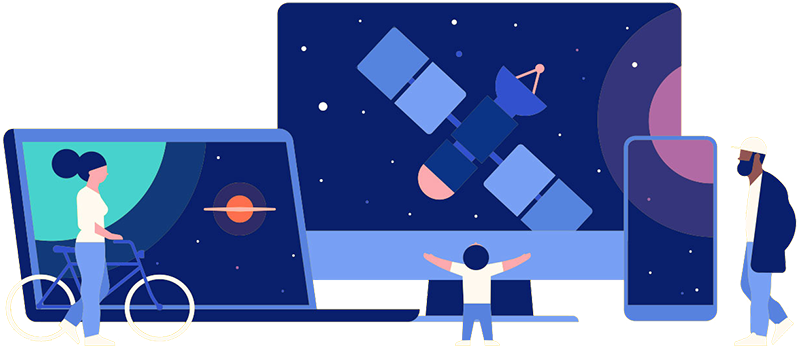
Online Access | Platform & Device Access | Cable or Satellite Access | Over-The-Air Access
Visit Access Guide
Need help accessing PBS Wisconsin anywhere?
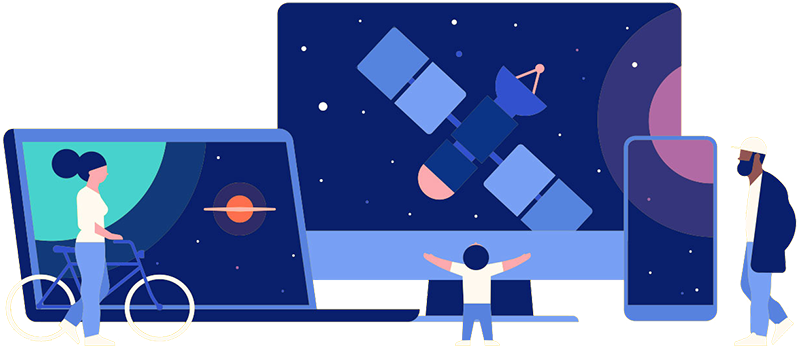
Visit Our
Live TV Access Guide
Online AccessPlatform & Device Access
Cable or Satellite Access
Over-The-Air Access
Visit Access Guide
Follow Us