Welcome everyone, Wednesday Nite at the Lab, I’m Tom Zinnen, I work here at the UW-Madison Biotechnology Center. I also work for UW-Extension Cooperative Extension, and on behalf of those folks and our other co-organizers, Wisconsin Public Television, The Wisconsin Alumni Association, and the UW-Madison Science Alliance, thanks again for coming to Wednesday Nite at The Lab. We do this every Wednesday night, 50 times a year. Tonight it’s my pleasure to introduce to you, Carsten Rott. He’s with the Wisconsin IceCube Particle Astrophysics Center and was born in Hannover, Germany and went to Gymnasium in Hannover, and got his undergraduate degree at the University of Hannover, Bachelor, or excuse me, Master’s Degree at Purdue University and his PhD at Purdue. Of course I always have to point out that Purdue is the French past participle for lost. (laughter) He also did for his work at Purdue, he worked at the Fermilab, which is the French participle for closed. (laughter) Then he went on to Penn State where he joined IceCube and then went to Ohio State and then he’s gone on to creative university that he will pronounce for us. And now he’s back here at UW Madison on a sabbatical. So I think he has covered four of the– Are there 12 universities in the Big 10, 14? 14, that’s why it’s called the Big 10, Tom.
So I hope you’re here to enjoying your time here in Madison. The lake will freeze over in a couple of months. You can go ice fishing. His talk tonight is on dark matter, “Don’t Be Afraid of the Dark,” and the cool thing is, the folks that are doing dark matter research are inviting us to consider October 31st every year not just as Halloween, but as Dark Matter Day, or as I would like to call it, Dark Matter Night. And so I think it’s great to have you here. I know nothing about dark matter. Let’s let Carsten tell us all about it. Please join me in welcoming Carsten Rott. (applause)
– Okay, thank you for the nice introduction and it’s great to see such a large crowd here to come out, to know what dark matter is. And I think I have to disappoint you because even after this talk, you will not know what dark matter is. (laughter) And the reason is that we don’t know yet what dark matter is. But the question about dark matter is actually one of the most fundamental questions that we are trying to answer. So since the early ages of humanity, people have been trying to answer the question what is the world made out of? And we have come a long way. So nowadays we know that everything in this room, pretty much, is made out of atoms. So, and we can also, we know that the atoms, we can decompose them in smaller particles so they’re made out of protons and neutrons and electrons. And even if you use a particle accelerator, you can break protons and you can find that protons are actually made out of quarks, which are the constituents that made up, that make up neutrons and protons. Now as we have built ever more powerful telescopes, we also started to observe the universe and we wondered what is the universe made out of? Is the universe also made out of the same matter that we have here, which we sometimes call the ordinary matter? So ordinary matter are the protons, neutrons and electrons and everything that we know about. So is the universe also made out of this ordinary matter? Now as we observed the universe, we came along evidence that actually the majority of matter in the universe is not the ordinary matter that we know of, but it’s dark matter.
And that’s actually why it is so important to answer the question “What is this dark matter?” Because in order to understand the universe, and to answer the question, “What is the world made out of?”, we have to understand what is dark matter. And we have actually plenty of evidence for dark matter, and I will, during this talk, explain to you what, how do we know about dark matter, what do we know about dark matter. And then I will come to the question, “How can we identify the properties of this dark matter?” And since this series is called Wednesday Nite at the Lab, I’ve actually brought a picture of my lab, which is the IceCube Neutrino Telescope, located at the geographic South Pole, and this is actually the laboratory that I work with, I mean not at, but I’ve been there a few times, but that’s only a few special occasions when we get to go there. And during this talk I will also explain you why we can use the IceCube Neutrino Telescope to look for dark matter and how we have searched for dark matter with IceCube. So now, let me begin with my talk and let me give you the overview. So this is basically what I’m gonna cover during the next hour here, so this is basically what I will suggest towards you. So let’s start with the first question, “What do we know about dark matter?” So we know from observations that the mass energy content of the universe, which is actually shown on the top right there, that the universe is made out of roughly five per cent ordinary matter, so these are the protons, neutrons and electrons, 27 per cent dark matter, and then 69 per cent dark energy. And I’m not going to talk about dark energy today, but that’s also definitely a very interesting question. So what we know about dark matter is namely that dark matter could be a new particle and basically all that we know so far about dark matter comes from the gravitational interaction that it has.
And maybe if you’re a fan of Star Wars, then you maybe remember this scene where Obi-Wan is trying to find a missing planet in the star charts. And basically he– He finds that the gravitational pull is pulling everything in this spot but there’s no star. So he concludes that, well, if the gravitational pull is there, then also a mass must be there. And this kind of resembles also what we know about dark matter. We know, everything that we know about dark matter comes basically from its gravitational interaction. So let’s go through some of the evidence that we have for dark matter and let’s start with rotation curves or reviewing, actually, the gravitational law. So you can see the picture here of Isaac Newton and his famous gravitational law, which is shown here, which basically shows that F, the force, that’s exerted between two masses, so in this case, the apple and the Earth, is given by the two masses and divided by the distanced squared times a constant G, which is a gravitational constant. So with this gravitational law, we can also, we can basically describe all the phenomena in our solar system and we can also use this gravitational law to predict how fast a planet has to move around the sun to stay on a circular orbit, and this is actually the plot here on the right side, and– Yeah, so you can basically see that all the planets follow this gravitational, or the prediction from the gravitational law. Maybe you think of Pluto, that Pluto is not a planet anymore, but of course, also the asteroids and everything else or dwarf planets in our solar system follow the same gravitational law.
Now, now we can actually ask, “Is this gravitational law universally true?” So if it works well in our solar system, does it actually work well also to describe the motion of stars in galaxies. So on the bottom right, you see a picture of a galaxy, so this is a large spiral galaxy, and this galaxy consists out of billions of stars. And in the middle you have basically a large mass concentrations and the stars are moving around this galactic center, basically. And the question we want to ask well, are the stars in the system following the gravitational law? So some of the, or the pioneering work on this actually has been done by Vera Rubin, Rubin, and she actually measured the rotational velocities of stars in in galaxies. She unfortunately passed away just about a year ago, which it was very unfortunate because, I mean, of course, it’s very unfortunate, but her, she did very, a lot of the pioneering work on this and also found a lot of the evidence for dark matter and she was also considered for the Nobel Prize and in particular, given that there are very few women who have won the Nobel Prize it would have been nice if she also received it. So actually let’s have a look at her work to honor her. Now she tried to measure galactic rotation curves. And to measure galactic rotation curves, she used the Doppler Effect. So we know the Doppler Effect basically from everyday life when you have a source of sound, for example.
So, let’s take this firetruck here. If the firetruck is moving towards you, the sound pitch will change then compared to when it’s moving away from you. And the same effect is actually present in light. So we know that light is also waves, and these waves actually get shifted, if the source is moving away from us or it’s moving towards us. And on the bottom here you can see an optical spectrum and you can see some absorption lines in there. And depending if the source is moving away or towards you, you will see that these absorption lines are shifting. And so basically if a source is moving away from you, it shifts more towards the red and if it’s moving towards you, it shifts towards the blue. So then on the right side you can see that she looked at galaxies for which we had basically an edge-on view and she then measured basically the color or she actually looked at specific spectral lines, so H-Alpha line, from hydrogen and she then basically could see how much the spectral lines are shifted. So out of this then she can derive what are actually the rotational velocities in this galaxy.
So this is a result that she found. She actually looked at many different systems but this is just one example. So on the right side you can see as function of the distance from this galactic center, the rotational velocity of the gas and stars in the system. And red is actually what you predict based on the gravitational law. And white is actually what she measured. So you can see that this gravitational curve actually stays pretty much flat. Which basically indicates that there’s more matter there than the luminous matter that we can see in this galaxy. And she did this measurement actually for many different galaxies, this is just some example of, yeah, some of the measurements that she did. So this is one case where we have found evidence for additional matter that we cannot see.
There’s also other evidence, for example, through gravitational lensing. So if you observe large clusters of galaxies, as I’ve shown here, and in the back you might have– You look at the distant galaxies behind that, you can see that the image can be lensed. And you can see this sort of– Let me see, where is the pointer? Okay, maybe it doesn’t appear, but– But you can sort of see this sort of ring shape, and these are actually galaxies behind this galaxy cluster and they get distorted and this distortion basically is based on the bending of the light due to the matter in between. And then we can actually check how much matter do we expect to be in between and how much bending do we actually see? And also from this you can derive that there is additional matter. Further evidence for dark matter and the, actually one of its fundamental properties, can be derived from an example which is called the bullet cluster. So this is actually a very beautiful image, but it’s actually a composite image that is kind of false colors, but let me just explain what you are seeing here. So this is an example where two galaxy clusters, so two clusters of galaxies are colliding and they– so they are collided and actually pass through each other. And what you can see on here in blue is actually where the mass is distributed. So this is actually from gravitational lensing, you can obtain a distribution where the mass is located.
And the red you can actually see where the hot gas is. So this is the ordinary matter. So when these galaxy clusters collided, actually a lot of the gas was stripped out and this is left in between, while the– most of the galaxies basically pass through each other. And the matter is basically located at this blue region. Now this actually tells us something about dark matter. Because we can see from this image that dark matter did not collide with each other, but just passed through each other. So from this we can actually derive this conclusion, at the bottom, that dark matter does not interact very strongly with matter and also not very strongly with itself. Other evidence for dark matter… comes actually from the structure in the universe.
You can actually see a movie up here which is a simulation of our universe and you can actually see how the structure in the universe is forming. So actually these distributions basically shows the distribution of the galaxies and matter in the universe and you can see that you basically get these filaments. So now– Let me actually move on to the next slide. I mean this actually goes on for quite a bit, the simulation. But if we actually look at the distribution of the galaxies in our universe we can actually find a structure that looks like this on the right. And on the left actually I have put different simulations of dark matter. And I labeled these– Well, I used different names: Cold, Warm and Hot Dark Matter. So DM stands for dark matter here. It actually describes how fast the dark matter particles are moving, so for example, if I think of a very fast- moving particle like a photon or light, which moves with the speed of light, then I would be in this regime of Hot Dark Matter.
And you see that the structure is very smeared out which means that dark matter cannot be hot and also cannot be warm but it’s cold and cold basically means that the dark matter is moving very slowly or the dark matter particles are moving very slowly. Okay, so then there’s further evidence for dark matter. Actually from imprints to the cosmic microwave background, maybe let me go quick on this. So this is actually radiation that’s emitted at the very beginning of the universe and this has been precisely measured actually with the Planck satellite and from this we can actually derive how much dark matter there is in the universe. Now let me move on. So one of the last evidence that I want to mention here for dark matter before I go to how we search for dark matter is actually evidence that comes from the clusters of galaxies. So in this image here, is the Coma Galaxy Cluster is shown. So each of these objects here is basically a galaxy and we can also, again, using this method from Vera Rubin, when she looks at the red shift or blue shift of these objects, we can look at the velocity, distribution of this object. And if you assume there’s more matter here than the velocity distribution of these galaxies will be increased, so they will be moving faster.
This is just basically what follows from gravity. Now this is actually when, in the 1930s, actually Fritz Zwicky, who was a famous astronomer from Switzerland who worked most of the time at Cal Tech, he actually observed this Coma Galaxy Cluster and he found basically the first evidence for this missing matter or how he called it, actually, dark matter, or he spoke German so he said Dunkle Materie, so the dark matter. So this is a picture of him and the Coma Cluster. So since these early days of Zwicky actually we have learned quite a lot about dark matter. So there’s conclusive, or there’s overwhelming evidence for dark matter on all scales in our universe as I have shown you, but the big question is still, what is this dark matter? So we have seen dark matter through its gravitational effect, but we want to know what is this dark matter. So let’s go try to answer the question, what is dark matter? Now from these observational evidence that I showed you, we can basically derive the following properties. So dark matter is cold, which means it’s, relative, the particles are relatively slowly moving. It’s neutral which means it’s not interacting through electromagnetic interactions. So it doesn’t absorb or emit light, because if it would emit light or absorb light then we would have easily seen it.
And it’s also not very strongly interacting with the ordinary matter. So this for example, we have seen in this example of the bullet cluster, where the two galaxy clusters are colliding. And also there’s this, this new particle has to be stable because if it decays, then it’s not around anymore. So these are basically the– everything that we know about dark matter. So then we can ask the question, well, is there any particle that we know of that has these properties? So now here I show a table of all the particles that we know of. So this is actually the Standard Model of Particle Physics, so this is basically what you can find or what has been derived based on years and years of studies at particle accelerators and colliders. And these are all the particles that we know of. And as you see on the top right, the Higgs Boson this was basically the last missing particle in this Standard Model of Particle Physics and we think now that this is basically complete. So we know all the particles that we have expected, we have observed.
And we can ask the question, well, is any particle in here, does it have the properties of dark matter? Well so first we have to ask the question are those particles stable? Or do they decay? And we can cross out actually a lot of them because most of them actually are very short-lived. So you can only produce them at the collider for a very short time and then they decay immediately. Also we said that dark matter does not emit or absorb light, so it’s not what we say electromagnetically interacting. So we can ask, okay, which of these particles are electromagnetically interacting so we can cross those out. So then with this, now we are only with three particles left, which are neutrinos but neutrinos are actually relativistic particles, so they move with the speed of light. So also we said dark matter should not be relativistic, because it should be cold. So we can also cross these out and there’s nothing left. So the only conclusion that we can draw, dark matter has to be a new particle, and– Well, it’s actually not so bad, I mean, to have a new particle, it just enlarges our horizon, maybe makes the world more interesting if there is a new particle and there are actually plenty of theoretical physicists who have cooked up models that predict, for example, new particles and just one of them is super symmetry which you might have heard about but– And people are looking for that. But there are also many other theories that predict new particles.
So now how can we actually look for this new particle? There are basically three different ways how we can search for dark matter. The first one is we can try to produce dark matter. So this is basically recreating the conditions that were present at the very early universe when it was very hot and dense, when we expect that dark matter was actually created, so. And we can do this actually at particle accelerator, so this is, or collider. So in this case we collide two particles with very high energy and produce, we can produce new particles. The second way how we can look for dark matter is actually through the scattering of the dark matter particle with ordinary matter. So this is the idea is basically like you’re playing pool, if you have an atom and you have a dark matter particle coming in, it could hit the atom in rare cases and maybe give some nuclear recoil and we can try to detect this interaction of dark matter particles scattering on an atom. And the last way we can look for dark matter, what you call dark matter annihilations. So when dark matter interact with each other, it could produce maybe standard model particles which we then can observe.
So now’s let’s have a quick look at these examples. The first one, which we call Make It! So the idea here is that we use two standard model particles that we collide at very high kinetic energies and then use this energy to produce new particles. Now this is being done at the Large Hadron Collider at CERN, near Geneva, Switzerland. And which is basically, yeah, 2.7, 27-ring circular accelerator in which we collide two protons and these protons are basically moving with the speed of light and are colliding and when such a collision happens this is basically shown on the top right here, many particles are created and out of these many particles that are created, we are trying to find a new particle. And at the bottom right is, or bottom left, is kind of such an example from kind of a theoretical prediction for some new particles that might be produced there. So analyzing all this data is incredibly difficult job and these experiments, which is for example, shown here, the CMS Detector, there are really thousands of people working on this and sorting through all this data. And after sorting through all the data, they have found basically no evidence for a new particle. I mean, they have found the Higgs boson, so there’s something, but there is no dark matter, or no physics beyond the standard model as we said. Now the second way how we can look for dark matter is to look for an interaction of dark matter with ordinary matter and this is what I call here Shake It!, so basically we shake the ordinary matter.
And actually outside here you can find a booth actually by Kim Palladino, so she’s sitting over there, and later on she can– Since she’s working on this dark matter direct detection, she can later also explain you more about this. But here I just want to give you the basic idea. So the basic idea is that this new particle which I noted by this Greek letter Xx (chi) here, is just hitting an ordinary atom and depositing some energy. We are trying to detect this small energy deposition. This is incredibly difficult to do because we know that dark matter is very rarely interacting so to run these experiments you actually have to go deep underground to be away from any background. So yeah, later on please check out the booth outside. Okay and then the last way, and this is actually the way that I mostly look for dark matter, is through indirect searches for dark matter. So in this case we assume that two dark matter particles hit each other and in this process, standard model particles are created and those particles we then are trying to observe. This is actually the basic idea here.
On the top left here you can see these two dark matter particles, or we call this WIMP dark matter here, weakly interacting massive particle, are hitting each other and then are producing some standard model particles and at the end you go through some decay chains, but at the end basically we can find stable particles that we can observe like light, or, yeah, neutrinos or some antimatter. And there are different experiments that are trying to look for this, or indirectly search for these signals. Now let me– I cannot go over all of them so let me just pick the experiments that I’m working on, which is the IceCube Neutrino Telescope. So this is actually a picture taken at the South Pole, so this is actually the counting house where all our electronics are located for this experiment. Now why have we constructed an experiment at the South Pole? Now, IceCube is a neutrino telescope and in order to detect neutrinos we need to make use of an effect which is called “shrink of light,” so this is actually when a neutrino interacts it produces some relativistic particles which then send out some shrink of light. And this is actually a picture of shrink of light. This is actually taken in a nuclear reactor. And in a nuclear reactor, you have also some relativistic particles produced that pass through the water and when they pass out through the water they send out his shrink of light. And basically we are trying to detect the same light but when a neutrino interacts with the ice.
Now the– Now in order to detect this light, we need a detector medium that has very good optical properties so that’s why actually we decided to build the detector at the South Pole. Because we can, at the geographic South Pole, the ice is roughly two miles thick and has very good optical properties, so that’s why we decided to build a neutrino detector there. So then the idea to detect neutrinos through the shrink of light emission can be explained in this following cartoon here. So you have a neutrino and that’s coming in on the bottom right here, it’s interacting, producing a particle which I call a muon here. And this muon is basically like a heavy electron. And when these neutrinos interact, in particular high energy neutrino, it produces a very energetic muon and this muon can actually travel several miles. And when it travels through the ice it sends out a cone of this shrink of light so all that we need now is a precisely-timed optical sensor array and with this precisely- timed optical sensor array we can then detect this shrink of light and reconstruct where the neutrino came from. Now this is a simulation here that actually shows this effect. So this, what you can see here are different strings, different strings of optical sensor modules and this, an energetic muon passing through this detector.
Let me actually show this again. And as it passes through the detector, you see this shrink of light that’s emitted and whenever this light gets detected by one of the optical sensor modules we mark this by one of these colored balls here which basically indicates the time actually when the optical module was hit. And the size of these blobs basically indicate how much light was detected. Now this IceCube Neutrino Telescope is actually a multipurpose experiment. It basically serves many different science or physics cases and basically we are trying to look for neutrinos of astrophysical origin. We’re trying to study cosmic rays, atmospheric neutrinos, even glaciology, trying to understand the ice and also look for dark matter. And of course, IceCube is headquartered here at UW-Madison, so maybe some of you know already a bit about it, so it’s very exciting experiment. And it’s actually a very large international collaboration. Also so there are about 300 people working on the experiment from all around the world.
From 12 different countries and all the countries are shown here. This is actually a picture of the South Pole where our experiment is located. This is actually the South Pole marker. This marker actually stands where the geographic South Pole is and you can– If you read on there, you can actually read this January 1st, 2008, so this is actually at the time when I was there, so I took that picture. This is actually, some image of me there. So at that time, the gray in the beard is actually frost, (laughter) but now it became real so time has passed on, so. Okay so then the detector is located very close to the geographic South Pole. You can see basically on the top right there, a map of Antarctica and where the geographic South Pole is located and on the– Yeah, basically you can see there where the geographic South Pole is and also where the Amundsen-Scott South Pole Station is. So this is actually where the scientists live.
Then there’s a Skiway, this is actually where we used to, where planes come in to bring in supplies and scientists to work there. And then very close to this station is actually where the IceCube Neutrino Telescope is located. And on the top left you can see actually a temperature chart and I haven’t seen any winters here in Madison yet, but I hear that during this time here in November, December, we go to yeah, maybe minus 30 degrees at the South Pole so maybe we’ll reach this temperature here also. But you can see from this temperature profile then in the austral summer, or austral winter period actually goes down to minus 70 or so, or even below that. And the IceCube detector was actually constructed during this period from November to February, so that’s actually when the South Pole is accessible. So after that, the station basically is not accessible and only a few people stay behind to keep the detector running. This was actually, on this picture here, the guy in the middle, tech, he was actually one of our Winterovers who stayed there one entire year to keep the detector running, so they are doing a tremendous job, actually. Okay, so on the, yeah, bottom right you can see kind of this footprint where the IceCube Neutrino Telescope is located. Now let’s actually go below the surface to see how the detector looks like.
And you can see here in the schematic view how the detector looks like. So first of all, the ice is roughly three kilometers deep and we have used a hot water drill to drill holes into this ice to a depth of 2.5 kilometers and then deployed optical sensor modules. So in the bottom right you can see actually such an optical sensor module. So it’s basically, kind of this basketball-sized module that contains a PMT or photomultiplier to basically a large light sensor, and with that, we want to detect the light from the neutrinos that are interacting in the ice. Then this detector was built over a period of seven years and has been taking data since 2011, ever since. Actually already during the construction period we obtained many very useful science data. Okay. This is how the detector would look like if you could float through the ice. These are actually the optical sensor modules.
So it’s basically at the bottom you can see this large optical or this large PMT and then also the top, it’s basically just some electronics that digitizes the signal that’s received by this optical sensor module. Okay, so then how do we look for dark matter with this neutrino telescope? Well one of the most spectacular ways actually how to look for dark matter with IceCube or very unique ways is actually to look for dark matter that’s captured in the sun. This is actually– It sounds at the beginning, quite out of this world that this would be possible but it’s actually a very reliable way to look for dark matter. And the idea is here that you have dark matter particles from the galactic dark matter halo, so as we said earlier, the galaxies are engulfed in dark matter and so these individual particles could scatter eventually on or with the hydrogen atom in the sun. When this scattering happens, they can lose some energy and they can become gravitationally bound to the sun. And this is basically what’s shown here in this pink line. So you have a particle that’s bound to the sun and, or this dark matter particle and it will maybe this orbit brings it through the sun and it has a higher chance of interacting again. It will lose more energy and eventually sink to the center of the sun. So if this process happens over millions of years, we basically accumulate in the center of the sun dark matter.
And when you have an enriched region of dark matter, eventually it will also interact with each other and when it interacts, it can produce the standard model particles. Now from all these particles that are produced in the center of the sun, they are basically no particles that could make it out, only neutrinos, are the only particles that can make it out of the sun. So neutrino, I should have also mentioned also called the Ghost Particles because they interact so rarely, so they can make it out of the sun and then come to the Earth. And if we detect such a neutrino with the IceCube Detector and see that the signal points back to the center of the sun, then this is a smoking gun signal for dark matter. Now we have performed such a search, actually several searches. This is actually some results from the scientific papers that we have written on this. Basically on the bottom left, you can see we are basically counting the number of neutrinos that we observe in direction of the sun. And this is actually what’s shown in these black dots here and this gray band is actually, maybe just look at one of the– yeah, one of this bottom region here, so. And this gray band is actually what we expect from a background from neutrinos that are naturally produced in the Earth’s atmosphere.
And you can see that this gray band which is kind of the natural background is basically flat and the dots that we observe are pretty much just scattered around it which means we don’t see any excess of events or any additional neutrinos coming from the center of the sun, so which is unfortunate, which means we have not seen any dark matter that’s captured in the sun. Otherwise, also if we would have seen it, for sure you would have heard about it already so. (laughter) And then on the bottom right, is actually the results plot, so this actually shows, and on the X axis, so on the horizontal axis, you have the math of the dark matter particle and on the vertical axis is basically the interaction cross-section or how strong this dark matter particle would interact with ordinary matter. And, this red line at the bottom, this is actually the result from the IceCube Neutrino Telescope, so it actually shows that the IceCube results are very relevant. So also on there are shown some other experiments and you can see that IceCube actually has some of the strongest bounds on dark matter, so we have not found dark matter, but we can still exclude some parameters space, which is then important for theorists, who are creating models for dark matter. To know kind of where there is no dark matter. So these searches are actually very important. There are many other ways also of how we have searched for, indirectly for dark matter. So we have looked for dark matter annihilation in our galaxy, in the galactic center, in small galaxies, in galaxy clusters. In all those we have not found any, any evidence for dark matter.
And similar, also other observatories observing with gamma rays, they have not found any, any evidence for dark matter. Now one of the things that we have, however, seen with IceCube recently is we observed very high-energy neutrinos. This is actually following this and also the completion of the IceCube Neutrino Telescope, we have also won this Breakthrough of the Year from Physics World and this is actually a very big or important discovery because for the first time we have observed energetic neutrinos from, yeah from, of extraterrestrial origin. So they are from somewhere from outer space. And we still don’t know where these neutrinos are coming from. So these neutrinos have humongous energies. So they actually have energies much higher than the energies that are created, for example, at the LHC, at the particle accelerator. Basically a thousand times higher in energy are these neutrinos or some of the neutrinos that we have observed. And the big question is where are these neutrinos coming from? So there are some natural astrophysical sources, source candidates like gamma ray bursts, active galactic nuclei.
But also people have suggested maybe there’s some new physics. So it could also be, and so we are also looking if these high energy neutrino flux has any exotic origin or origin from dark matter so. Okay so, with that, actually I’m come to the end and so let me conclude. So first of all, we know that most of the universe is made out of dark matter. So there’s overwhelming evidence for the existence of dark matter and I’ve showed you at the beginning of this talk the different ways how we have found the evidence for the existence of dark matter. So for example in large scale structure of the universe, the galactic rotation curves, through gravitational lensing, cosmic microwave background and so on. So there’s overwhelming evidence for the existence of dark matter on all scales in the universe. And we tend to believe that this dark matter is a new type of matter that we have– Yeah, a new type of matter and we have found or we have identified certain properties of it. So it has to be stable, it’s non-barionic, not very strongly interacting and it’s also not absorbing or emitting light and yeah.
It’s stable and not decaying. And to understand the universe, really, we have to understand or we have to learn what dark matter is and that’s why it’s such important, such, yeah, that’s why it’s so important to look for dark matter and all these– They’re intensive efforts now ongoing, by searches at particle accelerators indirectly through looking for the annihilation of dark matter and directly looking for the scattering of dark matter with nuclei and with, through all these searches we have– We were able to already exclude some of the models that have been proposed to explain dark matter but there’s still plenty of parameter space out there to explore and really we have to find dark matter to understand the universe and to answer the most, one of our most fundamental questions, what is the world made out of? And so I hope you enjoyed this talk and also please remember October 31st, Halloween, no. No, it’s Dark Matter Day, so that time you can learn more about dark matter so. Thank you for your attention. (applause)
Search University Place Episodes
Related Stories from PBS Wisconsin's Blog

Donate to sign up. Activate and sign in to Passport. It's that easy to help PBS Wisconsin serve your community through media that educates, inspires, and entertains.
Make your membership gift today
Only for new users: Activate Passport using your code or email address
Already a member?
Look up my account
Need some help? Go to FAQ or visit PBS Passport Help
Need help accessing PBS Wisconsin anywhere?
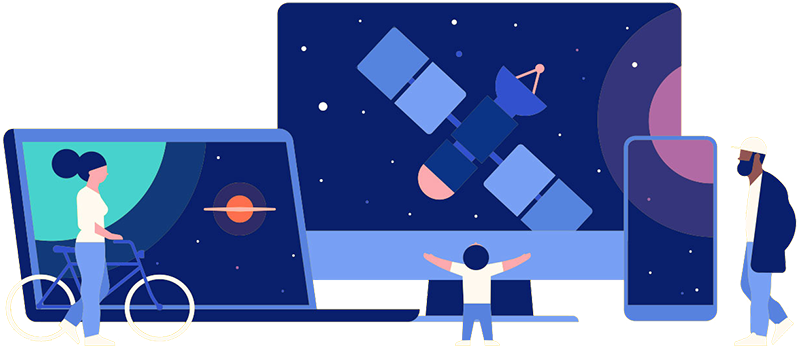
Online Access | Platform & Device Access | Cable or Satellite Access | Over-The-Air Access
Visit Access Guide
Need help accessing PBS Wisconsin anywhere?
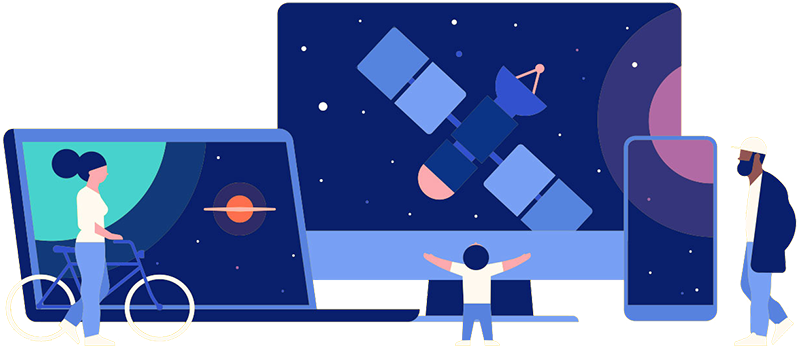
Visit Our
Live TV Access Guide
Online AccessPlatform & Device Access
Cable or Satellite Access
Over-The-Air Access
Visit Access Guide
Follow Us