– Welcome everyone to Wednesday Nite @ the Lab.
I’m Tom Zinnen.
I work at the University of Wisconsin-Madison Biotechnology Center.
I also work for the Division of Extension Wisconsin 4-H. And on behalf of those folks and our other co-organizers, PBS Wisconsin, the Wisconsin Alumni Association, and the UW-Madison Science Alliance, thanks again for coming to Wednesday Nite @ the Lab.
We do this every Wednesday night, 50 times a year.
Tonight, it’s my pleasure to introduce to you Michael Arnold.
He’s a professor in the Department of Material Science and Engineering here at UW-Madison’s College of Engineering.
He was born in Elk Grove Village in Illinois and went to Dundee-Crown High School there.
Then he went to the University of Illinois to study electrical engineering and then went to Northwestern University to get his PhD in engineering.
He did a postdoc at the University of Michigan and then came here in 2008 to join the faculty.
Tonight, he’s going to speak with us about how the Sun is ready to make electricity cheaper and greener here in Wisconsin.
Would you please join me in welcoming Michael Arnold to Wednesday Nite @ the Lab?
– Thank you, Tom.
I’m excited to have this opportunity to talk to you today about solar cells.
So my position at the university, I’m a professor at the university.
I both teach and do research and my research focuses on new materials, new semiconductors for solar cells and microelectronics.
And before we get started, I’d like to acknowledge briefly research support for our work on semiconductors and solar cells from the National Science Foundation, the Department of Energy, and the Department of Defense.
So I gave a presentation for PBS Wisconsin on the University Place series way back in 2010, also on solar cells.
But back then, my talk was a little bit more negative.
The title was, “Why Doesn’t My Electricity Come from the Sun?”
And the premise of the presentation was, well, everyone knows that solar electricity is this green, abundant energy source.
So why don’t we have it all on the roofs of our house and why don’t we get our electricity from sunlight?
Back then, the answer was, solar cells are just too expensive.
Well, in the more than one decade that has elapsed since 2010, there’s been a transformation in the industry surrounding solar cells.
And now, I’m here again to give a much more upbeat and much more positive presentation.
And my goal is to convince you that the Sun is ready to make your electricity cheaper and greener here in the Badger State.
So my presentation is gonna have a few parts to it.
First, I’m gonna try to teach the audience about our energy needs as a society and how much energy the Sun can provide us.
We use a lot of energy.
Can the Sun also provide us with a lot of energy?
And the answer is yes.
Then we’ll talk about how a solar cell works.
We’ll use very simple principles of chemistry and physics and engineering to understand how a solar cell converts sunlight to electricity.
We’ll talk about the different types of solar cells and how efficient they might be, their economics.
We’ll look at solar installations across Wisconsin, and we’ll talk about the future.
So let’s start by talking about energy and our energy needs.
So this is a flow diagram from the Lawrence Livermore National Laboratory that shows, from a recent year, a few years ago, 2019, our nation’s energy inputs and outputs.
So on the left are all the different sources of energy, and on the right are how we use it.
Now the numbers on this graph, which I’ll highlight more clearly in a second, are measures of energy used per year across the whole country.
The numbers are in units of quadrillions of BTUs.
So a BTU is one way of measuring energy.
And so you look at all the energy sources in our country that we use in a typical year, this adds up to around 100 quads or 100 quadrillion BTUs of energy.
Now another way to characterize energy or to quantify energy that’s used oftentimes in science is a joule.
And 100 quadrillion BTU is the same as 10 to the 20th joules.
So that’s 1 with 20 zeros after it.
That’s a lot of energy that we use here in the United States every year.
So among this energy, around 1.5% of that comes from solar right now, 8% from nuclear, a few percent from hydroelectric and wind.
And those green sources of energy amount to only around 10% to 15% of our current energy supply.
The balance comes from fossil fuels such as natural gas, coal, and oil.
Now, these energy sources are used across all the different parts of our society, residentially, commercially, industrially, and in transportation.
So we use a lot of energy, 10 to the 20th joules.
Next, I’m gonna try to help you understand just how big of a number that is.
So first, we’ll look at how that can be divided on a per person basis, a per capita basis.
So if we take 10 to the 20th joules and divide by roughly 300 million people in this country, that amounts to around 3 with 11 zeros behind it joules that each person on average uses per year in our country.
So how big is that?
How do we understand how 3 times 10 to the 11th joules really is?
So I have a question for you.
An analogy for you.
If you’re at the gym or lifting any heavy object like this 20-pound dumbbell, 20 pounds is pretty heavy.
Let’s say you have to lift that three feet.
How much energy are you expending against gravity to lift that weight one time by about three feet?
Well, the answer is only about 100 joules.
So this weight, lifting it once, pretty big task, 100 joules, and we are each using this much energy, 3 times 10 to the 11th joules per person every year.
So that is a lot of energy.
In fact, if we wanted to somehow supply our own energy by lifting this weight and then harnessing it and converting it to fuel or electricity, we would have to lift this weight, each of us, 340,000 times an hour, if we could harness that energy with 100% efficiency.
That would be every hour of every day of the entire year, and every person would have to be doing this.
So that’s just an enormous amount of energy.
Another way to think about energy is as a power.
So energy divided by time is power.
One joule per second is a watt.
Many of us are familiar with watts from light bulbs.
And an old-fashioned incandescent light bulb might use around 60 watts of energy.
A newer LED might use one-tenth that.
So if we take this 10 to the 20th joules per year and divide by the number of seconds in a year, which is around 31 million seconds, that equals 3 trillion watts.
So on average, at any given moment, our country is using 3 trillion watts of energy.
Worldwide, that amounts to around 18 trillion watts.
So we use a lot of energy.
Is the Sun a viable source for supplying a reasonable fraction of our energy needs?
And it is.
So the Sun is this massive object that’s emitting, in the form of light energy, an enormous amount of energy, 4 times 10 to the 26th watts of energy.
Now, of course, the Earth is 93 million miles from the Sun, and it’s only around 8,000 miles in diameter.
So it is only capturing a small fraction of the sunlight, but even still, the Earth is capturing around 3 times 10 to the 17th, or 3 with 17 zeros after it, watts.
So this is how many watts are being cast upon the Earth by the Sun, written out in full form at any given moment in time versus how much on average we’re using all across the world for all of our energy needs.
So you can see that the Sun is easily able to dwarf our energy needs.
If we could just harness a fraction of this energy, we could make a serious dent in our use of fossil fuels and replace it with this more green energy source.
Another way to think about solar energy is to take the total power and divide it by the area on the surface of the planet.
And so we receive around 1,000 watts per every meter by meter segments of land on a sunny day at around noon.
So of course, it’s not always sunny.
We have seasons, with more light in the summer and less in the winter.
And if you have clouds, then that can be reduced.
And so if you average across the entire year, we average at any given point on the planet somewhere between 100 and 300 watts for every meter by meter bit of land.
So of course, this will vary with where you are in the country.
In Arizona, which is where it’s much sunnier and further south, the average solar radiance is around 291 watts per meter square.
And we see about half that here in Wisconsin, mainly because it’s more cloudy.
So that’s the number of watts for every meter by meter bit of land.
And if you multiply the number of meters in Arizona or the number of meters square in Wisconsin, then this adds up to around 86 trillion watts of energy just hitting Arizona or 20 trillion watts of sunlight just hitting Wisconsin.
And remember, that’s the average amount averaging over night and day, cloudy and sunny, in all the seasons.
Nationally, we only use for all of our energy needs around 3 trillion watts of energy.
So we received more than enough energy just in Wisconsin to potentially power the entire country.
In fact, if you were to take a 140-by-140-mile bit of land out in the American Southwest and cover it with solar cells that were only around 20% efficient, which is easily technologically feasible, today, we could supply all of our nation’s energy needs.
Of course, that’s not practical right now because of challenges in transmitting that energy across the country.
But it goes to show that solar resources are enormous.
So humankind’s energy needs are very large, but the energy from the Sun is even more enormous.
And sunlight could power a large fraction of our society’s activities if the technology were available to make this possible.
And it is.
That technology are photovoltaic solar cells.
So the next part of the presentation will then shift to talking about how does a solar cell even work?
How does it convert sunlight to electricity?
So solar cell transduces light energy into electricity, and we’re gonna look at a solar cell first as a black box.
So our black box is gonna have two terminals, kind of like the terminals of a battery.
So you know that if you take a AAA battery, it has a positive side and a negative side.
Well, solar cell is very similar.
It has a positive side and a negative side.
In the dark, though, it provides no electricity, but you shine light on it, that generates positive and negative charges inside the solar cell.
The positive charges go to one electrode or one terminal of our solar cell and the negative charges go to the other terminal.
Then if you connect that solar cell to circuits, here showing a toaster oven, the electrons that are in one terminal go around the circuit and power our appliances, whatever it may be.
So what’s inside this black box?
How is the sunlight being transduced into electricity?
So at the heart of this black box is a material called a semiconductor.
Now, silicon is the most common semiconductor in the world.
Also, there are inactive components that offer structural support or the metal components that collect the charges, but the heart of it is a semiconductor.
So incidentally, the heart of a computer chip is also a semiconductor in which silicon is also the most common.
So computer chips and solar cells are both made from semiconductors.
So silicon is the most common semiconductor, as I said.
It’s very abundant in the Earth’s crust.
In fact, sand is an oxidized form of silicon.
Glass is largely comprised of silicon dioxide.
There’s a tremendous amount of silicon on the planet.
So we’re very fortunate in that regard.
There are other materials, combinations of elements from the periodic table that can also be semiconductors.
Many semiconductors, in fact.
For example, if you take gallium and arsenic and combine them into a compound, that is a semiconductor.
Cadmium, tellurium.
Copper, indium, gallium, selenium.
Even organic molecules made from carbon can be semiconducting, and combinations of organic molecules and inorganic compounds can be semiconductors as well.
So what makes a semiconductor different than, say, a metal or an insulator?
Well, semiconductor can act like either a conductor, like this copper wire, or an insulator, like we know that glass or rubber is electrically insulating.
And it could be switched dynamically to behave like either one.
A semiconductor could also undergo what’s called doping.
This is the addition of impurity atoms to our semiconductor, and that could make it conduct, when it is conducting, either positive charge or negative charge.
Furthermore, a semiconductor has a special property.
It has what’s called an energy gap, and I’m gonna explain what this means.
It’s also called a band gap.
And a band gap is the magic of how light is converted into electricity.
So to understand the band gap, we could kind of think back to high school chemistry class, where we learned about how electrons go around the positively-charged nucleus in an atom.
So what we learned in high school chemistry is a very simple picture of the atom, but it’s enough to understand the important principles of a solar cell.
So the electron that orbits closest to the nucleus, it’s kind of like Mercury orbiting around the Sun.
When the electron is in that orbit, it’s at the lowest possible energy.
That’s called the n=1 state.
And so on the right of this slide, the energies are separated vertically, with higher on the slide means higher energy.
If we look at an electron in the second shell, like Venus orbiting the Sun, that would be the n=2 electron, and that’s at higher energy.
So different electrons have different discrete energies in atoms.
Now, a material like a semiconductor is not just one atom in isolation.
It’s many atoms all packed together very, very closely.
And when the atoms come together very, very close, those electron orbits overlap with each other, and that causes splitting of the energy levels.
So on this slide, we’re looking at what happens when this splitting occurs.
We have one atom, and we’re looking here at just the n=1, like, the Mercury-like electron.
Later, we’ll look at all the different n=2 and n=3 electrons.
So if we look at n=1 electron, if we have one atom, we have one energy level.
But if we have two atoms, the electrons overlap and that n=1 energy level splits into two different energy levels.
If we have three atoms, it splits into three levels.
So if we have four atoms, it splits into four levels.
Now, a solid like a semiconductor has trillions and trillions and trillions of atoms.
And therefore, the energy level splits into trillions and trillions and trillions of different energy levels that are all so closely spaced that we could think of them as having a continuous band of energy levels.
So a semiconductor or any material for that matter has bands of energies, not discrete states.
So that’s the difference between an isolated atom and the material.
We have bands of states and materials.
So this splitting occurs for the n=1, the n=2, the n=3 levels, and then you count the number of electrons on each atom and you fill up the bands from the bottom up.
The bottom bands will be full of electrons and then the topmost bands will be empty.
If you have the situation where you have a full band, then a gap, and then an empty band, the gap between those is called the band gap, and that is measured in units of energy.
And what happens in a semiconductor is that when you shine light on the semiconductor, you kick up an electron from that full band up into the empty band that leaves a hole or the absence of an electron in the filled band.
So this generates now a positive and negative charge.
That’s what we want to happen in our solar cell.
We take light and now we have charge.
The next trick is we need to separate that charge to opposite sides of the solar cell.
So if you think back to that AAA battery, the positive terminals on one side and the negative charges on the other.
How do we get this positive negative charge separated is the next trick.
So this is a schematic of silicon atoms in a slab of semiconducting material.
Every silicon atom forms covalent bonds to four other silicon atoms.
Silicon is in the fourth column of the periodic table.
So it has four electrons in its outer shell.
So all the electrons go into bonding and sharing with neighboring silicon atoms.
There’s no extra electrons that are capable of moving around.
So in actuality, a pure piece of silicon has very low electrical conductivity because there are no electrons to move.
Now, when you shine light on the silicon, that generates an electron, and the positive charge, which is also called a hole, and we need them to separate.
The conductivity of the silicon has gone up.
But we can’t harness the energy of this light and the charge that had been generated from the light unless we could separate the charges spontaneously.
We need to coax those charges to separate on their own.
So the way that we coax charges to separate on their own is to utilize doping, which I have alluded to once before.
So doping of a semiconductor is not this type of doping.
So this is a photograph of the results of a race in the 2012 Olympics where many of the athletes later were caught to be doping.
So these athletes were injecting a foreign substance into the body to increase their performance.
Well, the doping of a semiconductor is very similar.
The doping of a semiconductor results from the intentional or unintentional addition of impurity atoms to change the performance of the material.
So dopants for a semiconductor mean taking other atoms from other places in the periodic table and mixing them in.
For silicon, the dopants are boron or phosphorus.
Other dopants are possible.
But these are the ones we’ll discuss as examples today.
For example, if you take silicon and you replace one of the silicon atoms with phosphorus, which is just to the right of silicon in the periodic table, phosphorus has five electrons.
Only four of those are needed to bond to the neighboring silicon, which means there’s one extra electron that’s free to move around.
This is called n-type doped silicon, electron typed, or negative doped silicon.
We could also do the same by adding boron atoms to our silicon.
Now, boron only has three electrons in the outer shells.
It’s to the left of silicon in the periodic table.
And when you add boron, it steals an electron from somewhere else so it can make four bonds to the silicon, leaving a hole.
So it has positive charge that can move around.
It’s called p-type or positive type or hole type doped silicone.
So we could make n-type doped silicon, p-type doped silicone, and the secret to making a solar cell is that you take each one of these types of silicon or semiconductor and you join them together to make what’s called a p-n junction.
Now, p-n junctions are common in diodes, in transistors, in computer chips, and they’re also the secret to making a photovoltaic solar cell.
So photovoltaic solar cell is a p-n junction.
Now, what happens is when you join the p and the n regions, some of these positive charges or holes then kind of migrates or move from the p side to the n side, and some of the electrons naturally want to move from the n side to the p side.
Now, the p side has a net negative charge ’cause some of the positive charges left and it’s added negative charge, and the n-side has a net positive charge.
It’s gained some of the positive holes and lost some of the electrons.
So the two different sides of the p-n junction become charged because of the diffusion of the free electrons and the free holes when we join the p-n junction together.
Now what happens is if you ignore all these dopants, the charge itself will induce the separation of new charges that are generated by light.
So light comes in, makes a positive and negative charge, and now because of the doping and the p-n junction, the charges automatically separate themselves.
And when they separate themselves, all the positive charges go to the p side, and all the negative charges go to the n side.
So this is exactly what we want to happen.
We’ve taken light, generated a charge, and now we’ve separated those charges to opposite sides of our device.
So a solar cell is this p-n junction, which is shown in the red and the gray layers of this schematic.
And then once the charges are separated, they’re collected with a grid of metal wires that then go to the positive and negative terminals of our solar cell.
On top of all this, there could be glass or anti-reflective coatings and other structural supports, but the heart of this type of device is this p-n junction.
So our black box is a p-n junction, shine light, you make charge, the charge is generated by exciting electron up in energy from one band to another, and then it’s able to go around the circuit.
So semiconductors are not just important for computer chips, but solar cells as well.
And the band gap affects what colors of lights a solar cell will absorb.
The band gap of most semiconductors used in solar cells is somewhere in the infrared so that we can absorb infrared lights and light higher energy such as visible and ultraviolet light as well.
Okay, so as I said in the introduction, I’m an engineering professor, so my goal there was to teach you some of the nuts and bolts of the physics and chemistry of solar cells.
Now, we’ll move more towards talking about some more practical aspects of solar cells.
And so if you want to forget all that chemistry and engineering, you could do so.
So let’s next talk about the types of solar cells and how efficient they are, and then move on to their economics.
And then practical things like solar installations in Wisconsin.
So this is a graph, a very busy graph from the National Renewable Energy Laboratory that’s showing the efficiency of solar cells on the vertical axis versus year.
Each line is a different type of solar cell being researched or developed by a university or company or national laboratory.
And this chart’s very interesting for a number of reasons, and I’ll kind of walk you through some of the important aspects.
And the first observation is that all these lines are tilted up.
So research and developments and just improvements in industry, in academia, in labs have resulted in more and more efficient solar cells over the years.
And the second observation is that the efficiency, which you read off the vertical axis, varies between around 10% to 47%.
So we take the energy from the Sun and we’re able to harvest between 10% to 47% of that as electricity.
Is that good?
So we’ve engineered with these p-n junctions this really cool technique for harvesting between 10% and roughly 50% of sunlight into energy.
Is that good compared to say, what biology can do?
So biology has had millions of years to evolve photosynthesis.
So let’s look at how efficient photosynthesis is.
So photosynthesis, of course, doesn’t generate electrical energy, but it takes light plus carbon dioxide and water and generates sugar and oxygen.
So the energy is being stored chemically in the form of sugar.
So this efficiency of this process is around 8.5%.
Now, of course, plant is gonna use some of that energy to restore and grow.
And so if we were to harvest, say, energy from plants, then we could harvest around 4% of that.
So we’ve already, in just a few decades of science and engineering, created artificial light-harvesting devices that are many times more efficient than what nature has accomplished in hundreds of millions of years.
So the efficiency is really pretty good.
So the other observation we can make from this NREL plot is that there are lots of different cell types, and I’ll kind of walk you through these.
Many of these are commercially available.
Some of these though are only available in the research lab.
So I’ll tell you which ones are the most relevant ones for you as a potential consumer of a solar cell.
First, I want to tell you though about some of the material science differences in these different classifications of cell to help you better understand them.
So one difference among different solar cell technologies is how the atoms in the semiconductors are arranged.
There are what’s called crystalline semiconductors, where all the atoms form a perfectly periodic pattern over the entire expanse of the solar panel.
There are polycrystalline semiconductors, in which there are multiple crystals, but they’re oriented at different directions and where the different crystals meet, there are disordered packings of the atoms.
And there’s amorphous solar cells, in which there is no long-range order whatsoever.
Generally, crystalline solar cells are more efficient, but they’re more expensive to make.
Commercial solar cells differ also in the number of p-n junctions there are in a single cell.
So that’s what’s called a single junction device, and that’s where there’s a single p-n junction.
Those are most common commercially, but it’s also possible to make multiple p-n junctions all stacked in series, each with a semiconductor with a different band gap that absorbs ideally a different color of light.
But those are more expensive to make, but can be a lot more efficient when they’re used in things like satellites, where price is really not as big of an issue as having as high of efficiency and as low as weight as possible.
Different cells also differ in the terms of the thickness of the semiconductor.
So silicon is a very thick crystal, but it’s also possible to make semiconductors from materials that absorb light much more strongly, and therefore you can use much less of that material in just a thin film.
And of course, different solar cells can be comprised of different atoms and compounds and elements across the periodic table.
So in this chart, the blue ones are the silicon technologies.
Those are the ones most common commercially.
Their efficiency is on the order of 24%.
We also have thin films; they’re in green.
It’s more strongly absorbing semiconductors, not based on silicon.
They can be cheaper to make, but they’re a little bit less efficient, maybe around 20%.
These purple ones are the multiple p-n junction cells.
They’re much more expensive.
They can be up to 47% efficient, but typically used in things like satellites.
And then the orange here is emerging materials, really only in the lab scale so far.
So let’s look at silicon and thin film some more.
So if you have solar cells on your roof or you pass a solar farm out in the field, these are the ones that you’ll see in real life.
So single crystal silicon solar cell, all the atoms are crystalline.
There’s a single p-n junction.
We have a thick slab of silicon some 100 micrometers thick, made in principle of silicon with those dopant atoms.
So these are made by taking, for example, sand, silicon dioxide, reducing that to pure silicon, so getting rid of the oxygen, purifying the silicon to get rid of unintentional dopant atoms.
So the silicon is over 99.999% pure.
That produces polycrystalline silicon.
That silicon then is melted at around 1,400 degrees Celsius, and seed crystal is dipped into the liquid silicon and pulled out, and as it cools, it solidifies into a single crystal, so in the upper right of this slide, there’s a person standing next to this huge, what’s called a boule of silicon.
All the atoms there have the same crystalline packing and there’s a single crystal in the orientation.
You then slice that boule up kind of like salami, into thin slices of salami to make silicon wafers that you can then dope and turn into p-n junctions and make your solar cells from panels, from these what’s called wafers of silicon.
There’s a nice video you could watch which I won’t show, but if you go to this address here, it’ll show you the whole process.
A PDF copy of my slides from this presentation is available on my website.
If you google “Michael Arnold wisc.edu” and go to the outreach page, you will find this PDF copy of the slides.
I’ll give an address at the end of the presentation as well.
So the single crystal silicon cells are one type of commercially available cell.
Polycrystalline is another.
Identical to single crystal silicon, but the silicon is not all the same crystal graphic orientation.
There’s multiple different crystals that come together with this order where different crystals join.
So the advantage of polycrystalline silicon is that you can make it at a much lower temperature.
So single crystal silicon, we are melting it at 1,400 degrees Celsius.
Processing materials at that high of a temperature is very expensive because it requires a lot of energy in and of itself, and there’s a high degree of perfection needed and it’s a very slow process.
So this makes the manufacturing of single crystal silicon solar cells much more expensive or more expensive than polycrystalline silicon.
So polycrystalline silicon can be fabricated at 650 degrees C from a gas.
So you take silane, which is silicon with four hydrogens and it produces hydrogen and silicon.
If you look at this photograph, what you see here are the different grains or the different crystals of silicon with different atomic orientations that have slightly different reflectivities.
And so if you see this grainy-looking panel out in the real world, you know it’s a polycrystalline cell.
And the last type of commercially available cell that you might encounter out in the real world is a thin film.
It’s what’s called a thin film cell.
These are polycrystalline single p-n junctions, very thin amounts of semiconductor on top of glass, and they’re made from elements such as cadmium and tellurium.
These could also be made at relatively lower temperatures such as 650 degrees C. This is a cross-sectional electron microscope image of what this cadmium tellurium solar cell would look like.
So the p-type semiconductor is cadmium tellurium, and in this case, cadmium sulfur is the n-type semiconductor that makes the p-n junction.
So among all these three different technologies, single crystalline silicon, polycrystalline silicon, and thin film such as cadmium tellurium, single crystal silicon is currently the dominant, commercially-used technology, but all are growing.
So this plot, the width of the plots is the global PV production, is a function of time, and you can see that this wedge is becoming wider and wider with time as the solar industry has grown exponentially.
All the different segments are growing, but single crystal silicon is growing the fastest.
And these cells are around 20% to 25% efficient commercially.
All right, so those are the different types of solar cells out there.
Probably you’ll encounter silicon solar cells if you were to come across cells in the real world.
So now let’s look at economics and how economics have dramatically changed over the last several decades.
So we’re gonna look at two different types of economics.
We’re gonna look at residential and utility scale, or solar farm scale.
So residential solar might be on the roof of a home.
A solar cell will output what’s called a direct current, which means that the electricity is constant with time, and that the currents and the voltage are constant with time.
These with the home, typically, appliances in the home typically use what’s called alternating currents of electricity, where the current and the voltage vary at about 60 hertz with time.
So in order to convert the constant electrical output of a solar cell to a time-varying output in a solar installation at the home, you also need what’s called an inverter.
So you have your solar cells on your roof, they’re connected to an inverter.
Then the solar electricity can be used by the home and excess could be sold back to the grid.
How a utility-scale solar farm would work would be you would have a large tract of land, you would have many solar cell modules and panels arrayed across the land.
There would be an inverter as well that converts the direct current to an alternating current.
And then this electricity would be transmitted over the existing infrastructure, the power grid, to residential homes, commercial homes, industrial locations, and so forth.
So this is a analysis of economics in terms of how much a solar panel will cost per watt.
So you could buy solar panels, and when you buy them, you might buy a thousand-watt panel, and what that number means, what the thousand-watt means is the peak power it could produce on a sunny day at noon.
It’s not the average power, but it’s kind of like the peak power.
And so one way to look at the economics of solar cells is you take the number of watts of your solar cell and take how much that would cost, and you take the ratio.
So the dollars per watts.
Of course, you have to pay not just for the panel, the solar cell itself, but you have to pay for its installation and other things.
And so this is an analysis by NREL of all the possible costs.
The panel, which is in yellow, the installation costs, which is in light orange, profit, which is in the black dashed lines.
And so this is really the actual cost to the consumer.
So if we look, around 2010, the cost of a solar panel for residential installation was around $7.50 per watt.
So in just one decade of time, this has plummeted to less than $3 per watt.
And since 2020, this continued to drop.
If we look at utility scale, which benefit from the economies of scale, we see the similar drop in price, but the absolute price started even lower and it’s ended lower even still.
So the price has dropped from $5 a watt to less than a dollar per watt at the utility scale.
So that’s one way to look at how the price of solar cells has dramatically decreased over time.
Another way is to look not at the cost per watt, but at the cost per kilowatt hour.
And this, to me, makes more sense because when you get your electricity bill every month, you see how many kilowatt hours you used, and the electricity company will charge you 15, 16 cents per kilowatt hour, and you can see what your electricity bill amounts to.
So how much energy you receive from your panel will depend on how many years it lasts.
And so this is a little bit more tricky of a calculation because you have to project ahead.
So solar panels that you can buy commercially typically have expected lifetimes upon the order of 30 years.
So there’s a large upfront cost to buy the panel, but once you’ve bought it and paid for its installation, it will provide, after that point, free electricity.
And the longer it lasts, then the more value you got for that panel.
So this plot is then analyzing the energy cost per kilowatt hour over a 30-year lifetime of a panel.
And it’s analyzing at three different locations in the U.S.
So we have of course different amounts of sunlight depending on where we are in the U.S. And so the more sunlight you get, you can get more electricity for the same cost of the solar panel.
So at the bottom of each bar is what the energy cost per kilowatt hour would be for Phoenix, the middle would be Kansas City, the top would be New York City.
In Wisconsin, Madison has about the same solar irradiance as New York City.
So if we look at the red data, that is without a tax credit.
Now, there’s recently, the U.S. government has created a tax credit for installed solar, both residentially and industrially.
And this tax credit will last for almost another 10 years.
And so the blue is the price after the tax credit.
So we could see that the cost has come down dramatically as a function of time in analyzing it this way, in terms of energy per kilowatt hour residentially, and also on the utility scale.
So if we look at the utility scale, even without any tax credit, the price has come down from 30 cents per kilowatt hour to less than 10 cents per kilowatt hour without tax credit.
And with a tax credit, less than five cents per kilowatt hour.
This compares to typical fossil fuel-based electrical rates on the order 15 to 16 cents per kilowatt hour currently.
So the price has come down dramatically over the last decade, and it continues to come down.
At the same time, the entire market for photovoltaics has increased exponentially.
So this is an analysis of the market between 1992 and 2018, and we see over 1,000 times growth in this industry.
So every 10 years, the industry is increasing its capacity by more than a factor of 10.
By 2018, reaching almost 1,000 gigawatts or a billion watts of installed solar across the globe.
If we look at the last 10 years on a linear scale, we see this exponential growth.
And this has happened not only internationally, but in the United States as well.
So by 2021, here in the U.S., we’ve reached 100 gigawatts of solar installations; that’s been spread across utility, commercial, and residential locations.
So why has there been this dramatic growth and dramatic decrease in price over the last decade-plus?
Firstly, capitalism and economies of scale.
So the business of manufacturing and installing solar cells has become dramatically more efficient.
Secondly, research and developments in the industry and in nationalized academia has increased solar cell efficiency, but more so, decreased materials processing costs.
And finally, government policy.
So I mentioned this tax credit.
So the Inflation Induction, Reduction Act of 2022 has extended this tax credit for solar, 30% credit through 2032.
So let’s look at the implications of all of this economics on solar in Wisconsin.
So I mentioned that the top of this bar graph represents energy costs per kilowatt hour here in Wisconsin.
Red is without tax credit and the blue is with tax credit.
So currently, we could expect around 15 cent per kilowatt hour electricity over 30 years without tax credit.
Nine cents per kilowatt hour with a tax credit.
So let’s think about this.
So if current fossil fuel-based electricity is on the order of 15 cents per kilowatt hour, that’s the price right now.
It will inflate with time over the next several decades, to much higher than 15 cents per kilowatt hour.
We could alternatively purchase photovoltaic solar cells or panels at a large upfront cost now, but then at that point on, we would receive essentially effectively free energy for 30 plus years.
So we’d be effectively locking in a price of only nine cents per kilowatt hour over the next 30 years.
So there would be a breakeven point around 15 years where we would repay the initial investment in panels.
And then after that, be making essentially a profit.
There is a very nice app on the internet that you could use to calculate all these numbers depending on where you live in Wisconsin.
It’s solarviews.com.
You type in the size of the number of kilowatts of panels you want and where you live and it will calculate then the price.
So if we wanted to install 5.8 kilowatts of installed solar on, say, the roof of our home, we would then pay a company to do that.
For installation and for their profit and for the panels themselves, around $10,000.
It then will tell us the payback period.
So that upfront cost would be effectively paid back in around 10 years, after which we would be making effectively a profit; we’ll gain free electricity.
So it’ll be a net profit of on the order of $20,000 to $30,000 over 30 years.
So in other words, an investment of $10,000 now would turn a profit of effectively $20,000 in around 30 years.
So we’re at the point, we’re at the junction where we can install solar to not just reduce pollution and because we’re interested in being green, but to save money as well.
So it’s a dramatic change from where we were in 2010.
The cost advantages of utility-scale solar are even better.
So if we look at utility-scale solar, the price is five cents per kilowatt hour in 2020, even without the tax credit.
So utilities can make money from these solar installations, and therefore, because money can be made, industry and utilities are building large-scale solar developments all across the state.
So here’s a list of current and upcoming installations across the state.
So we see over 1,000 megawatts of projects in construction or being planned.
So here’s an example of a recent one, the Two Creeks Solar installation in Manitowoc County.
This is 150 megawatts of solar panels.
It’s over 500,000 panels over 800 acres that are supplying energy for more than 30,000 homes.
Here’s another photograph of the Manitowoc installation.
Here’s another, the Badger Hollow Solar Park in Iowa County.
Again, 150 megawatts of installed or to-be installed panels.
That’s 500,000 panels over 1,000 acres.
Can power over 45,000 homes.
So let’s talk a little bit about the future.
So looking forward, economies of scale will continue to push down the price.
R & D of new materials and processes will continue to improve the dollar per watt ratio.
At some point, we’ll have so much installed solar across the state and across the the country that we won’t be able to use it all during the day when the Sun is shining.
And we’ll have to come up with more cost effective measures for storing the energy.
So for example, battery storage technology, so we could start harnessing solar electricity at night.
Well, the good news is that in the same way that there have been tremendous advances in the economics of solar cells, there have also been tremendous advances in the economics of batteries.
Another challenge with growing the solar industry here in Wisconsin is that we have a pretty well-established existing fossil fuel infrastructure already in place, and it’ll take time to transition and retire existing plants.
And another challenge of course is land usage.
We as a society have to decide how to best use lands, and there are many considerations that need to be made towards this end.
So I am a researcher.
So among all these things, what gets me most excited is the research and development of new materials for solar cells.
And there are lots of efforts towards this end.
We talked about mostly inorganic materials being used in solar cells, but it’s also possible to make solar cells from organic molecules and polymers that you could synthesize in a beaker at room temperature at very low cost.
My research is focused on using nano materials such as carbon nanotubes, which are shown in the upper right as semiconductors for very efficiently harvesting sunlight and converting it to electricity.
There are new inorganic semiconductors being developed and new inorganic-organic hybrid materials being developed for solar as well.
Just say something about this last one, the lower right, the inorganic-organic hybrids.
This is a new technology, a new material discovered just a few years ago.
This material can be made and turned into solar cells from solution at room temperature very inexpensively.
Already over 20% efficient solar cells have been made, which are almost as good as silicon cells.
The lifetime stability are still being researched and developed, though.
This is a hot area in research, if you happen to see a news article in the Popular Science press about some new material for solar cells, by very good chance could be these inorganic-organic hybrid perovskites.
So thank you very much.
I’d like to kind of wrap up by giving to you a few resources if you wanna learn more on this topic.
RENEW Wisconsin has an excellent website talking about many of the issues we talked about today.
So does Solar Energy Industries Association.
If you wanna learn more about how a solar cell works and the basics of the physics and the science, you can see this Department of Energy page.
You can find a copy of these slides at arnold.engr.wisc.edu/ outreach.html.
So thank you so much.
Solar technology has rapidly matured, the scale of solar manufacturing has grown exponentially.
Price has plummeted.
Solar is now cost-competitive or cost-superior.
The Sun is now ready to make your electricity cheaper and greener here in Wisconsin.
Thank you.
Search University Place Episodes
Related Stories from PBS Wisconsin's Blog

Donate to sign up. Activate and sign in to Passport. It's that easy to help PBS Wisconsin serve your community through media that educates, inspires, and entertains.
Make your membership gift today
Only for new users: Activate Passport using your code or email address
Already a member?
Look up my account
Need some help? Go to FAQ or visit PBS Passport Help
Need help accessing PBS Wisconsin anywhere?
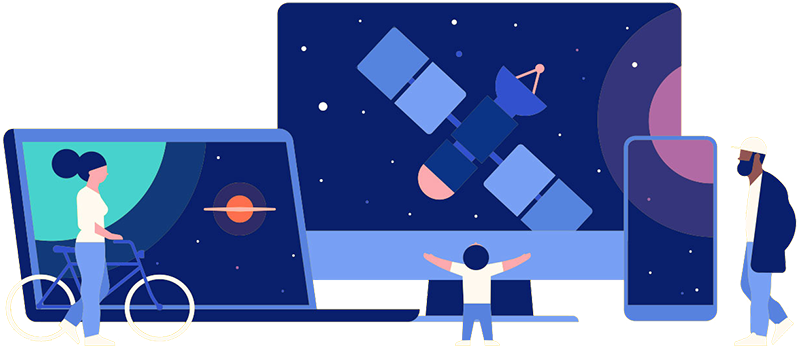
Online Access | Platform & Device Access | Cable or Satellite Access | Over-The-Air Access
Visit Access Guide
Need help accessing PBS Wisconsin anywhere?
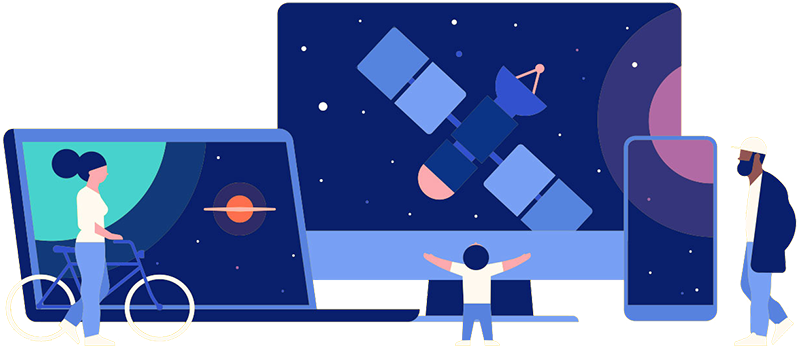
Visit Our
Live TV Access Guide
Online AccessPlatform & Device Access
Cable or Satellite Access
Over-The-Air Access
Visit Access Guide
Follow Us